the Creative Commons Attribution 4.0 License.
the Creative Commons Attribution 4.0 License.
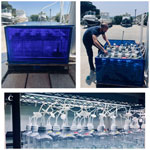
Laboratory experiments in ocean alkalinity enhancement research
Maria D. Iglesias-Rodríguez
Rosalind E. M. Rickaby
Arvind Singh
James A. Gately
Recent concern about the consequences of continuing increases in atmospheric CO2 as a key heat-trapping agent (Wuebbles et al., 2017; Masson-Delmotte et al., 2021) has prompted ocean experts to come together to discuss how to provide science-based solutions. Ocean alkalinity enhancement (OAE) is being considered not only as an ocean carbon dioxide removal (CDR) approach but also as a potential way to mitigate ocean acidification. Over the last 2 decades, inter-laboratory comparisons have proven valuable in evaluating the reliability of methodologies associated with sampling and analysis of carbonate chemistry parameters, which have been routinely used in ocean acidification research. Given the complexity of processes and mechanisms related to ecosystem responses to OAE, consolidating protocols to ensure compatibility across studies is fundamental for synthesis and upscaling analysis. This chapter provides an overview of best practice in OAE laboratory experimentation and facilitates awareness of the importance of applying standardized methods to promote data re-use, inter-lab comparisons, meta-analysis and transparency. This chapter provides the reader with the tools to (1) identify the criteria to achieve the best laboratory practice and experimental design, (2) provide guidance on the selection of response variables for various purposes (physiological, biogeochemical, ecological, evolutionary) for inter-lab comparisons, (3) offer recommendation for a minimum set of variables that should be sampled and propose additional variables critical for different types of synthesis and upscaling, and (4) identify protocols for standardized measurements of response variables. Key recommendations include ensuring reproducibility through appropriate experimental design and replication, assessing alkalinity thresholds for secondary precipitates for each experimental approach and condition, using recommended targets of alkalinity (3000–4000 µmol kg−1) and levels exceeding these concentrations to mimic responses at the site of deployment/non-equilibrium and to use intermediate alkalinity levels to identify potential nonlinear responses, and establishing the appropriate experimental design to address questions at specific levels of organization (chemical, physiological, molecular) and assuming different scenarios (e.g., mimicking impacts at the site of deployment in a non-equilibrated system versus steady-state scenarios in an equilibrated system).
- Article
(3796 KB) - Full-text XML
- BibTeX
- EndNote
Laboratory studies need to be reproducible, consistent and transparent (Box 1) to provide the scientific community and regulators with useful information to move the field forward and facilitate the development of safe guidelines. Based on numerous modeling studies, ocean alkalinity enhancement (OAE) appears to be a promising ocean carbon dioxide removal (CDR) approach, with the likely beneficial side effect of mitigating ocean acidification (Burt et al., 2021; Hartmann et al., 2023; NASEM, 2022; Wang et al., 2023). Laboratory experiments are urgently needed to determine the CDR potential of various OAE methods as well as OAE impacts at various levels of biological organization (ecological, physiological, biochemical, molecular). The emerging empirical studies offer insight while revealing gaps in our knowledge of the mechanisms governing OAE and its effect on marine biota (e.g., Ferderer et al., 2022; Gately et al., 2023; Yang et al., 2023). For example, the conditions preventing or limiting the formation of secondary precipitates and the pros and cons of various alkali are still under debate. Given that empirical work on OAE is still in its infancy and that some of the assumptions based on modeling studies remain untested, this chapter is an evolving document that will be updated as the OAE community continues to release results.
Laboratory manipulations allow making observations in a highly controlled environment using model species or subsets of populations (selected species or populations). Results are generally considered highly reproducible (Box 1) and therefore laboratory manipulations are viewed as a necessary step to either generate hypotheses to test in the field or vice versa, when field experimentation is an option. Under the latter, field observations guide the laboratory experiments to validate field results in well-known systems and under tightly controlled conditions.
A number of approaches – batch, semi-continuous and continuous cultures – have been used to address diverse OAE settings (e.g., at the point of deployment, under steady-state conditions, air- versus non-air-equilibrated seawater) and various biological scenarios (specific stages of growth, life cycle and abrupt/short-term versus long-term responses to manipulations). In some cases, specific stages during the life cycle of organisms can be selected (for example, larval versus adult stage, sexual versus asexual phase). Time series laboratory experiments are less restricted than mesocosm experiments with regards to the duration of experiments because they tend to be “cleaner”, with relatively low bacterial numbers and generally without biologically confounding factors (viruses, predation, competition for resources, etc.). Therefore, the cause–effect relationships are easier to elucidate as conditions and organisms can be tested in relative isolation, and there is the possibility of extensive replication.
The main limitation of laboratory experiments is that the dynamic phenomena occurring in the natural environment cannot be captured in the laboratory and, therefore, results may not be applicable to real life scenarios. For example, in laboratory experiments the influence of mixing processes, conditions governing particle flocculation or the linkage to higher levels of biological organization (e.g., predation) are difficult to discern (see Forbes and Calow, 2002; Martin et al., 2014). Portable lab experiments, such as deck incubations aboard research vessels or outdoor incubations, with some influence from the local environment (e.g., diurnal alterations of light, water flow through from the coast to maintain in situ temperature) as well as community-level mesocosm experiments, are the conduit to field manipulations. These large-scale community experimental tanks address the importance of the physico-chemical conditions, space, density-dependent effects, biotic interactions and the complexity of natural environments in their response to OAE manipulations/buffering, or boosting, and the direct effects of environmental stress on organisms (Paiva et al., 2021).
This chapter provides best-practice guidelines in OAE laboratory experimentation and offers recommendations to enable data re-use, inter-lab comparisons and transparency. We offer recommendations regarding (1) the criteria to achieve the best laboratory practice and experimental design; (2) the selection of response variables for various purposes (physiological, biogeochemical, ecological, evolutionary) for inter-lab comparisons; (3) a minimum set of variables that should be sampled and additional variables critical for different types of synthesis and upscaling; and (4) protocols for standardized measurements of response variables.
The rich insights obtained into ocean acidification research are key to supporting OAE studies. However, as crucial as it is to follow guidelines when designing laboratory experiments, it is equally important to acknowledge that there may be potential confounders and challenges that may not be accounted for in the guidelines. Being able to conduct quantitative laboratory intercomparisons, including interspecies comparisons, will be critically dependent on identifying recommendations regarding experimental design, sample collection and data analysis. Important considerations include the source of alkalinity, rate of alkalinity addition, testing of air-CO2-equilibrated versus non-equilibrated seawater, and the effect of ancillary variables (e.g., temperature) in multifactorial experiments which are known to yield complex and variable results (e.g., see the interactive effects of ocean acidification and warming – Harvey et al., 2013). The guidelines provided in this chapter should significantly improve the quality and impact of the OAE research, which is required to meet the identified societal need for research on OAE and other types of ocean CDR (NASEM, 2022).
An exploration of procedures, patterns and challenges associated with ocean acidification research has offered ideas on how to design rigorous and reproducible laboratory experiments that enable measuring and monitoring carbonate chemistry shifts and biological responses to ocean acidification (Cornwall and Hurd, 2016). Cornwall and Hurd (2016) reported that 95 % of the experimental work between 1993 and 2014 had interdependent treatment replicates or lacked replication in clearly defined treatments or did not report sufficient methodological detail. More broadly, results from Wernberg et al. (2012) from marine climate change experiments between 2000 and 2009 reported that ∼ 49 % of the experiments had identifiable issues with their experimental procedures, and 91 % of the experiments reported showed a lack of treatment replication or pseudo-replication. Amongst the studies, 9 % included extreme/unrealistic treatments of temperature or pH far beyond worst-case scenario projections (Wernberg et al., 2012), although “extreme” pH/alkalinity conditions may prove useful to define thresholds of tolerance and upper limits of alkalinity enhancement and to understand underlying physiological mechanisms of acclimation to alkalinization. While the urgent need for field trials requires careful consideration of treatment levels, in order to maximize the insight gained from OAE experiments, testing conditions outside the year 2100 IPCC CO2 emission scenarios are encouraged. These conditions outside worst-case scenario projections will further our knowledge on the mechanisms governing biological (e.g., shell production) and abiotic (e.g., particle aggregation, secondary precipitation) responses to applied chemical CDR.
Like in ocean acidification research, careful attention should be given to the advantages and disadvantages that concern the choices of dissolved inorganic carbon species to be measured and to how error propagation will affect the calculated parameters (Martz et al., 2015). Moreover, dissolved organic matter (DOM) is known to contribute to alkalinity (Kim and Lee, 2009; Koeve et al., 2010), although the presence of strong acidic groups in organic matter can decrease net alkalinity (Hu, 2020; Middelburg et al., 2020). Depending on the type of system under investigation, attention should be paid to whether to apply titration alkalinity (typically used in ocean studies) versus the charge balance approach (often used in freshwater systems, with high concentrations of dissolved organic matter) (see Middelburg et al., 2020). Results from ocean acidification mesocosm experiments focused on phytoplankton revealed that nutrient-limited communities appeared to be more responsive to changing carbonate chemistry than those having access to high inorganic nutrient concentrations (see Paul et al., 2015; Sala et al., 2016; Bach et al., 2016). These observations indicate that trophic state might play a role in the susceptibility of organisms to the changes in carbonate chemistry driven by alkalinization. Also, competition between species has been found to be altered under various carbonate chemistry conditions (see Kroeker et al., 2013a), which merits a focus on experiments that address preferential selection of taxonomic groups under different alkalinity conditions. Although applying nutrient-limiting conditions is experimentally challenging, understanding how species succession and community composition might respond to alkalinization could in part be addressed in a laboratory context.
While it is fairly straightforward to determine how individual changes in parameters influence chemical and biological responses, understanding the impacts of multiple parameters (e.g., increased alkalinity and warming, increased alkalinity and resource availability, such as nutrients, light or prey) can be challenging as they can interact in complex ways. Indeed, ocean acidification research has revealed antagonistic, synergistic and additive responses when studying ocean acidification and warming (Byrne and Przeslawski, 2013; Kroeker et al., 2013b; Harvey et al., 2013; Pistevos et al., 2017). Identifying tipping points and interactive effects when other parameters (e.g., temperature) are altered in seawater, in addition to alkalinity, is critical given the capacity of these parameters to drive (otherwise unpredictable) shifts in species abundances, biodiversity and community composition, physiological outputs, survival, and reproduction (Crain et al., 2008; Darling and Côté, 2008; Galic et al., 2018).
The different steps in experimental design are outlined in Table 1. The process starts with natural or artificially made seawater with or without nutrient additions. One must consider whether adding nutrients/food/prey is required; for example, whether exploring OAE impacts is intended in conjunction with specific scenarios, e.g., nutrient fertilization, specific stages of growth or population development, and the extent to which nutrient additions or any other basic manipulation of the environmental conditions might impact the interpretation of results. For OAE manipulations where sterilization is required for the experimental setup, autoclaving is discouraged given the alterations in carbonate chemistry, including the loss of CO2, leading to a decrease in dissolved inorganic carbon and alterations in alkalinity (increase with increasing salinity/decrease with precipitation of carbonate) triggered by autoclaving. Instead, filter sterilization of seawater through small-pore-size filters (e.g., 0.22 µm filters) is required to remove particles and most bacteria and produce the stock media where different manipulations are applied to create different alkalinity treatments.
Table 1Experimental considerations for OAE experimentation. Medium preparation: the seawater can be obtained from coastal or open-ocean sites. Filtered seawater or, when appropriate (e.g., when growing autotrophic organisms), seawater supplemented with nutrients, for example, using or variations of media (see Guillard and Ryther, 1962), will be used for growing organisms. Seawater media can also be prepared from artificial recipes (e.g., Aquil medium; Morel et al., 1979) when specific compounds or elements need to be altered in seawater. Media must be sterilized by filtration rather than through autoclaving, and nutrients can be added, typically from stock solutions. When possible, moderate aeration should be applied. Types of alkali include adding pulverized mineral directly to the media and promoting dissolution physically (e.g., by stirring); dissolving the mineral separately and filtering out any particles remaining in the media before experimentation; dissolving salts to mimic the chemistry of the dissolved alkali (e.g., to mimic limestone dissolution and dissolve CaCl2 and NaCO3, which result in higher dissolution rates); and adding liquid alkali such as NaOH. Establishing time series prior to the experiment to determine time frames regarding the length of experiment, frequency of sampling, etc., is recommended. Experimental design: in addition to optimizing reproducibility by designing enough replication and testing the reproducibility of the method, researchers should remain engaged with respect to protocols and experimental design to avoid artifacts and undesirable side effects of the methodology. When possible, ensure the equilibration of seawater gases with air and define experimental time frames to test impacts under conditions representative of the site of deployment (where limited gas exchange occurs) and those representative of steady-state/equilibrated conditions. Although most laboratory experiments address short-term impacts, chronic effects can be tested in long-term incubations. Sampling and analysis: the parameters to be considered should allow inter-lab comparisons, address functional properties of organisms (e.g., calcification, silicification, particulate organic carbon) and fulfill needs to improve model parameterizations. It is important to establish well-defined time windows for sampling as well as the frequency of sampling to capture the physical, chemical and biological properties of the studied system. It is advisable to limit the time of sample storage to minimize observations that might confound the interpretation of results (e.g., reverse weathering during storage). Stock solutions (e.g., nutrient and alkalinity solutions) must be stored in the appropriate vessels to avoid contamination from leachates coming out of the vessel itself (e.g., silicate contamination from solutions stored in borosilicate containers). Detection limits and accuracy and precision should be offered for each protocol.
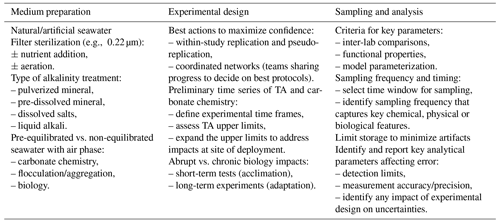
There are several approaches to simulating the addition of alkalinity that capture different components of any manipulation experiment. The first approach could be testing the impact of the instantaneous addition of alkalinity to seawater to mimic the impact on seawater chemistry and ecosystems at the point of deployment. The second involves aeration and equilibration with the atmosphere to explore the physico-chemical response to a steady-state/equilibrated scenario. In the latter instance, the medium is aliquoted out to the experimental vessels/tanks where aeration is applied to promote air equilibration. Monitoring carbonate chemistry through time enables determining when the equilibration of seawater with air occurs.
3.1 Sources of alkalinity
As yet, it is unclear what the optimal method or source of alkalinity enhancement may be in order to simulate the desired chemistry in seawater media. Proposed sources of alkalinity include silicate minerals (olivine, basalt), brucite, limestone and its derivatives (quicklime and portlandite), NaOH, and mine tailings (NASEM, 2022; Nawaz et al., 2023). Given the slow dissolution kinetics of the minerals, generating alkaline solutions artificially is acceptable. For example, Gately et al. (2023) simulated alkalinity enhancement via a limestone-inspired solution by adding Na2CO3 and CaCl2 or its hydrated form (CaCl2H4O2) to seawater. Adding Na2CO3 raises total alkalinity (TA) and dissolved inorganic carbon (DIC) at a 2 : 1 ratio, with 2 mol of TA added by two conservative Na+ ions in Na2CO3 and 1 mol DIC added by CO. CaCl2 does not raise alkalinity because it adds equal amounts of positive and negative conservative charge to the solution from Ca2+ and 2 × Cl−. However, it does raise the calcium in solution and therefore the saturation state of the seawater with respect to CaCO3.
Many possibilities for solid or liquid alkalinity additions are being considered (see OAE Guide 23, Chap. 3). While adding minerals as precursors of alkalinity can provide a source of potentially beneficial nutrients (e.g., silicate, iron, magnesium) (Hartmann et al., 2023), the possible toxic effect of metals leached out of minerals, an example being nickel (Ni) leached from olivine (Montserrat et al., 2017), is of concern. The use of NaOH is currently gaining attention given that its environmental footprint is perceived as smaller than the mining of alkaline minerals, which necessitate an expansion of mining operations, transportation and industrial processing, which are energetically costly and can lead to air pollution. Additionally, the amount of Na added to seawater is very small relative to the large background of NaCl in seawater.
The addition of NaOH and other forms of alkalinity to seawater cause initial spikes in pH and a drop in aqueous CO2 that can be balanced to a steady state via bubbling with air (Table 1). Determining abiotic and biotic responses to the initial spikes in pH and drops in CO2 is an important step in addition to understanding responses under steady-state conditions. It may be that large manipulations of alkalinity are needed to elicit a measurable and reproducible response, and the required alkalinity concentrations will be refined with more detailed modeling but, based on current information, proposed targets for alkalinity manipulations are 3000–4000 µmol kg−1 (Renforth and Henderson, 2017). The concentration of alkalinity (∼ 4000 and ∼ 3000 µmol kg−1) expected at locations in the ocean where alkalinity is initially added is the concentration of alkalinity expected once ocean circulation has dispersed the alkalinity over a larger area (Renforth and Henderson, 2017). Alkalinity thresholds for the formation of precipitates will need to be determined for each experimental approach and condition. It is, however, recommended that researchers consider using alkalinities exceeding the recommended targets and utilize intermediate treatments (e.g., 2000, 4000, 7000 µatm kg−1 seawater) rather than just low/high treatments, in order to identify potential nonlinear and even parabolic responses. This approach has led to important and unexpected outcomes in ocean acidification research (e.g., Ries et al., 2009).
3.2 Impacts of impurities/metal leachates
An important consideration in OAE studies is the impact of metals leached from dissolving minerals and their ecotoxicological potential on marine organisms. For example, although some elements (e.g., Fe and Mg) leached out of minerals could be beneficial micronutrients, the potentially toxic effect of metals such as nickel (Ni) (Montserrat et al., 2017), leached from olivine, is of concern. Diverse responses have, however, been reported with respect to Ni, and it appears that some cyanobacteria rely on Ni more than other photosynthetic organisms (see Dupont et al., 2008, 2010; Ho, 2013). A recent laboratory study testing olivine leachates (containing Si, Ni, Mg, Fe, Cr and Co) in phytoplankton revealed either positive or neutral physiological short-term responses in all treatments (Hutchins et al., 2023). However, one should consider the role of long-term experiments to examine organismal and population adaptation of metal exposure as well as potential bioaccumulation and biomagnification impacts in consumers.
Another important consideration is the effect of pH on metal speciation as pH and a change in the concentration of OH− and CO ions can affect the solubility, adsorption, toxicity and rates of redox processes of metals in seawater thus altering the interactions of metals with marine organisms (Millero et al., 2009). When dissolving minerals in seawater, one must consider non-stoichiometry and incomplete dissolution, perhaps as a result of dissolution of impurities, precipitation of secondary minerals, or preferential leaching of elements from the mineral surface (Brantley et al., 2008; NASEM, 2022). The formation of secondary precipitates has been observed in several studies exploring the dissolution of olivine (Fuhr et al., 2022) and limestone derivatives (Moras et al., 2022; Gately et al., 2023; Hartmann et al., 2023). Using an alkaline solution rather than reactive alkaline particles has been recommended to reduce carbonate precipitation unless seawater critical supersaturation levels are exceeded (Hartmann et al., 2023). In addition, runaway CaCO3 precipitation, a condition where more alkalinity is removed than initially added, reduces the OAE CO2 uptake efficiency. More complex precipitates containing Fe, Si and P were observed in a study using a limestone-inspired OAE approach revealing that mineral precipitation caused by seawater alkalinization can also remove inorganic nutrients from solution (Gately et al., 2023).
Maintaining alkalinity following OAE is critically dependent on the carbonate saturation state, its temporal evolution and particle surface processes (Hartmann et al., 2023). To minimize the loss of alkalinity and maximize alkalinity enhancement, Hartmann et al. (2023) propose the application of an alkaline solution in CO2 equilibrium with the atmosphere and/or solutions with tested saturation levels to prevent a further increase in supersaturation and the precipitation of carbonate “to avoid loss of alkalinity”. A separate reservoir where alkaline solutions have been prepared is desirable for testing the upper limits of alkalinity addition and identifying saturation thresholds to minimize precipitation.
4.1 Experimental replication
Replication is important to determine if results are reproducible, although one must consider that when results are so dependent on precise experimental conditions that replicability is needed for reproducibility, the result may be unique and potentially less relevant than a phenomenon that can be reproduced by a variety of independent, non-identical approaches (see Casadevall and Fang, 2010). A number of experimental designs can be used to achieve adequate statistical replication (Fig. 1). For example, simple replication involves experimental units (each of the replicates) per treatment where all the conditions are manipulated independently but in the same way for that treatment and where responses to the treatment are measured (defined by Hurlbert (2009) as the “evaluation unit”) and each experimental unit can be regarded as independent. In temporal replication, multiple measurements are made through time (temporal trends) on the same experimental unit. Sacrificial replication involves the use of multiple sampling times per treatment (for example, a time series) and multiple experimental units at the time of sampling. Each approach has distinct strengths and limitations, and the choice of the approach depends on the scientific questions and the extent of the risk of error propagation. For example, one might choose sacrificial replication for certain chemical manipulations that require sampling from vessels with comparable volumes but choose instead temporal replication for monitoring the evolution of a microbial culture or the physiology of fish over time under certain alkalinity conditions.
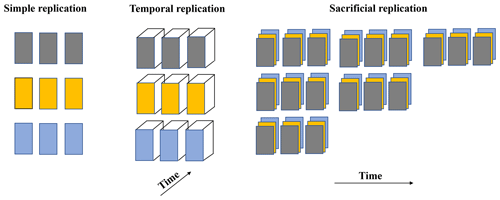
Figure 1Examples of experimental laboratory design with regards to replication. Each treatment, represented by a color, contains experimental units (replicates). Each experimental unit is treated as an independent experiment except in the sacrificial replication approach, where each replicate is treated statistically as an experimental unit.
4.2 Preliminary experiments
In addition to testing the biological responses to abrupt enhanced alkalinity, marine organisms can be exposed to enhanced alkalinity conditions after the equilibration of seawater pCO2 with that in the air phase following alkalinity addition. Ideally, aeration should be maintained to ensure O2 levels required by marine animals and also maintain stable pCO2 levels in the alkalinity perturbation experiments. Depending on the organism tested (a few organisms do not tolerate aeration in tanks), aeration might or might not remain for the duration of the experiment (Table 1). The vessels used in OAE experiments might not be traditional tanks used in aquaria but rather any type of container adequate for different types of organisms (e.g., culture flasks for bacteria, conical flasks, carboys for phytoplankton, open tanks for echinoderms and fish) with air lines to introduce aeration into the media. When running multifactorial experiments (e.g., temperature and alkalinity), designing an analysis plan and concrete experimental questions to interrogate can help determine the sample size and minimum number of treatments.
An analogy to OAE is the use of lime soda and other alkali to combat acid rain, which has caused deleterious changes in freshwater ecosystems for more than half a century in northern Europe and North America. To reverse some of these' changes a number of governmental and nongovernmental teams have applied lime and other neutralizing compounds to streams, rivers, lakes and catchments in the most affected or most ecologically valuable regions (see Clair and Hindar, 2005). Another example is the effects of seawater buffering mainly by the addition of Na2CO3 addition utilized by the commercial shellfish industry (e.g., Ragg et al., 2019), which showed a broad improvement in larval health compared to undersaturated waters.
Standardizing technical details in protocols, sampling, sample processing and analyses is crucial to control for variation introduced by reagents, sample storage and other factors. The collection and curation of metadata associated with each sample are critical for data interpretation, inter-lab comparison and drawing conclusions to move forward with planning field deployments for research purposes. For studies involving more than one level of biological organization, i.e., grazing experiments and competition experiments, particular attention should be paid to designing adequate controls.
The effects of OAE and its interactions with other parameters might differ depending on the duration of the experiments. Indeed, in ocean acidification studies, compensatory metabolic pathways appear to take longer to become established, depending on factors such as the exposure history (Calosi et al., 2013) and phase of the life cycle (Hettinger et al., 2012). In a study testing ocean acidification and warming, biological effects were not detectable in the short term but were instead manifested over time (Godbold and Solan, 2013). It was suggested that species responses to seasonal variations in environmental conditions might explain these differences that, depending upon timing, can either exacerbate or buffer the long-term directional effects of climatic forcing (Godbold and Solan, 2013).
4.3 Recommended minimum set of variables to report
To improve comparability between future work, we recommend a minimum set of variables with the understanding that more variables might be added as new results emerge (Table 2). We recommend measuring and reporting at least the following variables (shown in bold in Table 2).
-
At least alkalinity and one more parameter of the carbonate system must be measured to calculate key carbonate chemistry parameters including bicarbonate and carbonate ions, CO2, pH, and the saturation state of CaCO3 polymorphs. This information is critical to determine chemical alterations in the dissolved inorganic carbon system as a result of alkalinization.
-
Resource availability (e.g., prey, dissolved inorganic nutrients, light) are needed to monitor the growth conditions.
-
Particulate organic carbon (POC), nitrogen (PON) and phosphorous (POP) are required to learn about trends in biomass production and stoichiometry.
-
Basic physiological properties (respiration, photosynthesis) should be measured to inform biogeochemical models and learn about biologically mediated fluxes of elements.
-
Some functional group-specific properties, particularly those involving mineral precipitation (calcification, silicification) and those with environmental effects (e.g., toxin production) and with climate-relevant impacts (nitrogen fixation/denitrification) in context-specific cases must be measured.
-
The size of offspring and fecundity rates can be used as indicators of trans-generational plasticity and adaptation to alkalinization.
Table 2Examples of responses to ocean alkalinity enhancement to be measured in experimental manipulation studies. Knowledge need: M – medium; H – high. Measurement mode: MM – manual mode; S – sensor; SD – sensor in development. A minimum variable set is highlighted in bold. Selected references are provided as examples of protocols.
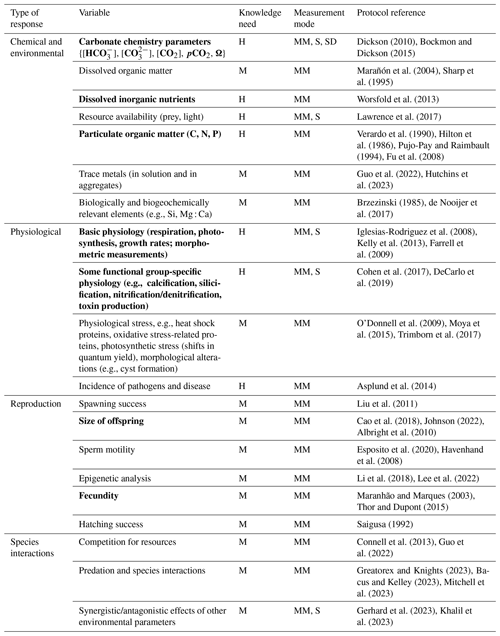
Other variables are important in the exploration of specific questions such as the following: how does seawater alkalinization affect biodiversity, how does metal bioavailability change under increased pH and what is the role of organic alkalinity in coastal systems? The variables and protocols listed in this chapter are not exhaustive and only provides a proxy sample largely based on the literature on climate impacts on marine systems and ocean acidification.
4.4 Type of experiments
Laboratory experiments can be designed to both address short-term responses and to explore the longer-term adaptation to chronic exposure to enhanced alkalinity conditions. Filtered natural seawater should be used, when possible, in incubations unless artificial seawater is required (for example, when studying the effect of metal concentrations). Short-term manipulations involve the use of batch, semi-continuous and continuous incubation experiments. In batch incubation experiments, all resources are provided at the beginning of the incubation, without further addition, and sampling takes place during a short time period (hours, days, weeks). Only gases and alkali can be added during the course of the experiment. When biological processes are measured, a phase during the life cycle (e.g., larva/adult, vegetative cells/gametes) or growth (healthy, exponentially growing/resource-limited, stationary growing organisms/senescent organisms) is typically targeted. Sampling is conducted until the nutrients are consumed and beyond that if decaying populations are the focus of the investigation.
Given that resources (light, nutrients) are the limiting factor in batch incubation experiments, the organisms are in the exponential growth phase for a limited time period. To expand sampling and replication during the exponential growth phase, resupply of nutrients using a semi-continuous culturing approach can prevent food/nutrients from becoming a limiting factor. When the studied organism is phototrophic, one must ensure subculturing (microbial cultures) or appropriate arrangement or organisms to prevent light limitation. The advantage of semi-continuous culturing is that it allows investigating trends over extended time periods, increasing replication and higher yield. Generally, the resource is added manually or pumped from the nutrient supply vessel into the culture vessel during exponential growth or when specific conditions are met (e.g., when a certain biomass concentration is reached).
In continuous cultures, the rate of the addition of nutrients is controlled to maintain steady-state cell growth. This system is known as chemostat, where, typically, a volume of culture medium is added and the same volume is removed from the growing culture. A challenge with this type of “bioreactors” is that, over long time periods, they can be more susceptible to microbial contamination and long-term phenotypic and genotypic variance in the cultures (Reusch, 2014).
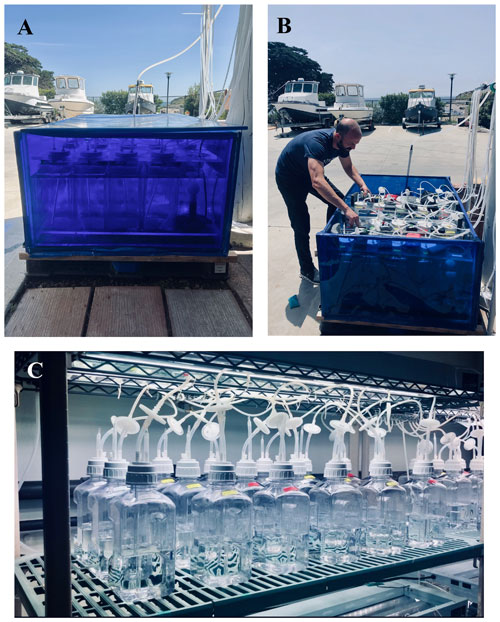
Figure 2(a, b) Portable incubator with blue filters to adjust photosynthetically active radiation (PAR). A scalar PAR sensor (LI-COR) can be observed within the incubator (a, right side). (c) Laboratory experiment using aeration and sacrificial replication. Images were taken by James Gately (a, c) and Sylvia Kim (b).
Portable incubation experiments that simulate regional in situ alkalinity deployments are an important step in understanding seawater alkalinization and its impact on marine organisms prior to field testing. This type of incubation experiments, which simulate alkalinity additions under diverse local in situ parameters (e.g., temperature, irradiance, nutrients), can be accomplished using portable incubators on board research vessels (i.e., deck incubations) or outdoors, at coastal research facilities (Fig. 2).
When studying photosynthetic organisms, high-quality light filters should be attached to the acrylic tank to adjust photosynthetically active radiation (PAR) within the incubator (e.g., Fig. 2). To maintain in situ seawater temperatures, an inflow port can supply seawater to the incubator. Effort should be taken to ensure movement of seawater quickly through the incubator to maintain a uniform temperature.
When collecting natural seawater, one must consider how biological interactions (e.g., grazing) could confound results and filter accordingly. Unlike laboratory experiment, which allow for seawater–air-phase CO2 equilibration, portable incubation experiments require instantaneous alkalinity additions; thus, careful consideration should be given to the method of alkalinity addition used. When adding liquid alkalinity, e.g., solutions (e.g., 1 M) of NaOH one must consider that flocculation commonly occurs upon alkalinity addition (Subhas et al., 2022). Adding pulverized minerals directly to the treatment vessels is another option, although this method may yield incomplete dissolution or slow dissolution (e.g., Fuhr et al., 2022) with undesirable effects including secondary precipitation, particle aggregation and detrimental biological impacts (NASEM, 2022). Some researchers have opted for mimicking mineral dissolution instead (see Gately et al., 2023). As in the traditional laboratory experiments described above, vessels within the incubator should ideally be aerated during experimentation. In addition to chemical and biological parameters, PAR and temperature data should be collected throughout the experimental time frame through discrete sampling or semi-continuously using sensors and data loggers. The best practices outlined in Box 1 should be adhered to when planning portable incubation experiments. Effort should be taken to position the incubator in a way that avoids confounding factors such as light contamination (e.g., from the ship).
Technical variability amongst experimental methods ranging from sampling and sample processing can propagate through the various steps before analysis; for example, chemical analysis and molecular work/sequencing can be error-prone (e.g., Catlett et al., 2020). The use of blanks every time sampling is conducted is essential for detecting contamination originating from the experiment itself or from the adjacent environment (e.g., exogenous sources such as surface contamination, flagellates in droplets through aeration). When possible, several barriers to contamination are recommended (e.g., filters at various points of aeration). Additionally, for samples (other than those preserved for analysis of alkalinity, dissolved inorganic carbon analysis or pH) that are kept for further analyses, contaminants that grow during shipping or while samples are being stored can sometimes be reduced by freezing at −80 ∘C, when possible, or by using the appropriate preservatives when storing at ambient temperature is required (e.g., ethanol, paraformaldehyde, glutaraldehyde). Attention should be paid to the material of vessels where samples and solutions are stored; for example, avoid borosilicate bottles to store nutrients or alkalinity solutions as silicate can be leached into solution.
Establishing time series prior to the experiment to determine time frames regarding the appropriate length of the experiment and frequency of sampling is recommended. It is important to establish well-defined time windows for sampling as well as frequency of sampling to capture physical, chemical and biological properties of the studied system. It is advisable to limit the time of sample storage to minimize observations that might confound the interpretation of results (e.g., reverse weathering during storage) (Subhas et al., 2022).
5.1 Criteria for key parameters
For the most part, laboratory experiments are aimed at elucidating the physiological performance and biogeochemical responses of organisms (rather than communities) to physical or chemical alterations in the environment, although conclusions about responses in ecological fitness could be drawn from laboratory experiments (Table 2). Importantly, environmental change can affect species differently and interactions between species that are sensitive to environmental change can function as ecological leverage points through which modest changes in abiotic conditions are amplified into large changes in marine ecosystems (see Kroeker and Sanford, 2022). These interactions can be measured as competition, predation and symbiotic relationships (mutualism, commensalism and parasitism) that can vary along environmental gradients that cause stress (Stachowick, 2001; Bruno et al., 2003; Ma et al., 2023).
Criteria for selection of species should include whether the organism is amenable to laboratory experimentation, the amount of background knowledge on the organism's physiology and biogeochemistry, ecological importance of the organism, and local and global impacts. Considerations when selecting organisms should also include geographic origin (e.g., temperate/tropical/polar) and ecosystem type (e.g., benthic vs. pelagic). Special attention should be paid to those species that (1) significantly impact or respond biogeochemically to chemical changes caused by alkalinity addition (e.g., possibly calcifiers, photosynthetic organisms), (2) keystone organisms (e.g., corals, salmon, sea stars, toxin-producing phytoplankton), and (3) organisms/functional groups of known vulnerability to climate change (corals, urchins).
Calcium-carbonate-producing organisms are particularly interesting because of their known sensitivity to changes in carbonate chemistry and because any alteration in their abundance or calcification rates could have implications in the CDR potential of alkalinization. The mineralogical composition of carbonate-containing organisms might possibly be affected by alkalinization. For example, recent meta-analysis of studies exploring the effects of the carbonate chemistry shifts caused by ocean acidification revealed effects on shell state, development and growth rate (Figuerola et al., 2021). Biomineralization studies should explore species-specific responses driven by mineralogical composition (calcite, aragonitic, high/low Mg calcite) of their tests, shells and skeletons. Environmental and biological control on calcification, particularly any changes in the Mg content in calcite driven by the use of brucite and other minerals potentially adding Mg to calcite, must be reported, as calcite with a high Mg content is less stable in aqueous solutions (Ries et al., 2016). Empirical studies have shown that the Mg Ca ratio of Mg-calcite-producing organisms generally varies proportionally with seawater Mg Ca (e.g., Ries, 2004, 2006), and therefore particular attention should be paid to the Mg content (and solubility) of biomineralized calcite. The addition to proposed Ca- and Mg-containing minerals – Ca(OH)2 (slaked lime), Mg(OH)2 (brucite), CaCO3 (limestone) or (Mg,Ca)CO3 (dolomite) – will alter the Mg Ca ratio of the seawater. An extensive body of literature reports biogenic and abiotic precipitation of low-Mg calcite when seawater Mg Ca falls within the calcite stability field (seawater molar Mg Ca < 2) and the biogenic and abiogenic precipitation of aragonite and high-Mg calcite when seawater Mg Ca falls within the aragonite stability field (seawater molar Mg Ca > 2) (Ries, 2010). Thus, modification of local seawater Mg Ca ratios by OAE has the potential to favor aragonite and high-Mg calcite organisms if seawater Mg Ca is increased and low-Mg calcite organisms if seawater Mg Ca is decreased. This is an important area of future OAE research.
Central to OAE laboratory experimentation is our ability to measure any possible stress induced by alkalinization and learn about underlying mechanisms behind acclimation to the chemical alterations of seawater caused by OAE. This can be achieved by measuring basic functions (growth rates, size, reproductive success); sensitivities to alkalinization might be organism-specific and possibly trophic-level-specific (e.g., Voigt et al., 2003; Gilman et al., 2010), although most laboratory experiments do not address the complexity of trophic interactions. Similarly, measuring adaptation and diversity in acclimation between and within related organisms is a challenge, and the ocean acidification literature has revealed how important it is to pay attention to diversity of responses (see Kroeker et al., 2010).
Stress is often measured as a reduction in organismal performance or fitness caused by environmental change (Schulte, 2014). In addition to these general physiological or behavioral responses, markers of stress such as oxidative stress are often used. For example, it is well established that the production of reactive oxygen species (ROS) can increase due to environmental stress including ocean acidification (Lesser, 2006; Lushchak, 2011). Many biomarkers are commonly used for studying oxidative stress in marine organisms (Cailleaud et al., 2007; Vehmaa et al., 2013), and an increase in ROS and superoxide dismutase and catalase activities has been reported in marine animals under stress (von Weissenberg et al., 2022). Heat shock proteins (HSPs) are also used as molecular markers of stress because of their abundance, high sensitivity to stress and ubiquitous expression (Gross, 2004). Among all HSPs, HSP70s are the most studied as a strong up-regulation of HSP70 production has been demonstrated broadly with the exception of Hydra oligactis (Bosch et al., 1988) and some Antarctic animals (La Terza et al., 2001; Place and Hofmann, 2005).
5.2 Measurements of nutrient uptake rates
The uptake rate of carbon and other nutrients that results in the observed standing stocks of particulate matter involves many physiological processes that are sensitive to changes in inorganic carbon chemistry and pH (Matsumoto et al., 2020). Chemical changes following the addition of alkalinity might alter physiological processes that represent sources (calcification, respiration) and sinks (photosynthesis) of CO2. One should also pay attention to the reciprocal interactions between these physiological processes and the chemically altered environment as even minor changes in biological processes or in the balance between them can have implications for the CDR potential and biodiversity.
One of the most unknown effects of OAE is the fate of biological fixation rates of different elements (e.g., carbon and N2 fixation rates). Such rates are measured in batch cultures and bioassay (mixed natural community) incubation experiments (LaRoche et al., 2010). While the objective of culture experiments is to understand the effect of environmental parameters on the elemental uptake by particular species in a lab, bioassay experiments have to deal with a rather complex species interaction in the field or after subsampling of mesocosms in a lab (Hutchins et al., 2007; Paul et al., 2016). Labeled/enriched (∼ 99 %) stable isotope tracers represents the most used method for rate estimation these days. The rate calculation is based on isotopic mass balance equation (Montoya et al., 1996):
where [POM] is the concentration of element of interest (C or N) at the end of the incubation. Likewise, Af = atom % in POM at the end of incubation, A0 = atom % in POM at the start of the incubation, t is time of incubation and Ae is the isotopic enrichment in the dissolved form after the tracer addition at the start of the incubation.
This equation/method is sensitive to analytical protocols in routine incubations (White et al., 2020) and might be even more sensitive in OAE incubations due to the issue of gas equilibration in tightly capped bottles. While the C substrate-based incubations are supposedly straightforward, N2 gas incubations face the challenge of under-equilibration leading to underestimation of rates. But OAE incubations can produce larger errors in the C fixation estimates as well. This is because NaHCO3 is generally used as a C substrate. To estimate 13C isotopic enrichment after tracer addition (term in Eq. 1), a DIC value is normally assumed (as it does not change much at a given region). But OAE is expected to increase (or fluctuate) DIC during the experimental period, and thus a measured DIC value should be used in the enrichment factor calculation. Likewise, the 14C method, which is widely used for marine primary production and calcification rate measurements due to its sensitivity (Nielsen, 1952), also requires treatment-specific determination of DIC concentrations. Likewise, slow dissolution of N2 gas poses a challenge to accurately estimating isotopic enrichment factor (Ae), and it is advisable to measure this term.
Although the analytical precision of C and N isotopes is of the order of sub-per-mil levels, many times the low reported rates (< 0.1 nmol N L−1 d−1) are questionable (Gradoville et al., 2017). Therefore, the detection limit of rate measurements and its proper reporting is a major concern. To overcome this, following the propagation of analytical and statistical errors in each term of mass balance equation (1), Gradoville et al. (2017) have proposed to report minimal quantifiable rates (MQRs) and the limit of detection (LOD) in triplicate samples. We ought to follow these protocols in the rates measured in OAE. In addition, we must make sure to sample/filter sufficient water to achieve 35 µg N and 150 µg C in the sample for reliable mass spectrometric measurements.
The field of OAE faces a great diversity of challenges given the continuously evolving experimental approaches and emerging data availability that will undoubtedly provide new information and ideas to optimize best practice in laboratory experimentation. This chapter highlights the need for attention to the design, sampling, performance and analysis of laboratory procedures used in OAE laboratory experiments. The criteria we present to achieve best practice in laboratory experimentation and design focus on reproducibility, factors affecting CDR potential and organism health (e.g., alkalinity conditions leading to flocculation, aggregation), establishing suitable experimental controls, and identifying the appropriate level of biological organization (physiological, molecular) to study biotic responses to OAE. Key response variables providing information on alterations in seawater chemistry following alkalinization, growth of organisms/biomass buildup/reproductive success and biogeochemically relevant properties (e.g., photosynthesis, respiration, calcification) under elevated alkalinity conditions should be measured and reported. The main recommendations include the following:
-
Ensure reproducibility through appropriate experimental design and replication.
-
Determine alkalinity thresholds for the formation of precipitates for each experimental approach and condition.
-
In addition to the proposed alkalinity target values of 3000–4000 µmol kg−1 (Renforth and Henderson, 2017), use concentrations exceeding these recommended values to mimic responses at the site of deployment/non-equilibrium and use intermediate alkalinity levels to identify potential nonlinear responses.
-
Establish an appropriate experimental design to address questions at specific levels of organization (chemical, physiological, molecular) and assuming different scenarios (e.g., mimicking impacts at the site of deployment in a non-equilibrated system versus steady-state scenarios in an equilibrated system).
Given the emerging nature of ocean alkalinity enhancement as a research field, this chapter will evolve to update guidelines as more results become publicly available. Frequent assessments of knowledge acquired from emerging and future studies and a review of best practices are needed to keep the OAE community engaged and forward-thinking.
No data sets were used in this article.
MDIR wrote the original manuscript with contributions from the authors. REMR, AS and JAG contributed with the critical review, commentary and revision of sections and general comments on the paper.
Competing interests are declared in a summary for the entire volume at: https://sp.copernicus.org/articles/sp-oae2023-ci-summary.zip.
Publisher’s note: Copernicus Publications remains neutral with regard to jurisdictional claims made in the text, published maps, institutional affiliations, or any other geographical representation in this paper. While Copernicus Publications makes every effort to include appropriate place names, the final responsibility lies with the authors.
We thank Ocean Acidification and other ocean Changes – Impacts and Solutions (OACIS), an initiative of the Prince Albert II of Monaco Foundation, for its support throughout the project. We extend our gratitude to the Villefranche Oceanographic Laboratory for supporting the meeting of the lead authors in January 2023.
This research has been supported by the ClimateWorks Foundation (grant no. 22-0296) and the Prince Albert II of Monaco Foundation.
This paper was edited by Jean-Pierre Gattuso and reviewed by Justin Ries, Alex Poulton, and one anonymous referee.
Albright, R., Mason, B., Miller, M., and Langdon, C.: Ocean acidification compromises recruitment success of the threatened Caribbean coral Acropora palmata, P. Natl. Acad. Sci. USA, 107, 20400–20404, https://doi.org/10.1073/pnas.1007273107, 2010.
Asplund, M. E., Baden, S. P., Russ, S., Ellis, R. P., Gong, N., and Hernroth, B. E.: Ocean acidification and host–pathogen interactions: blue mussels, Mytilus edulis, encountering Vibrio tubiashii, Environ. Microbiol., 16, 1029–1039, https://doi.org/10.1111/1462-2920.12307, 2014.
Bach, L. T., Taucher, J., Boxhammer, T., Ludwig, A., The Kristineberg KOSMOS Consortium, Achterberg, E. P., Algueró-Muñiz, M., Anderson, L. G., Bellworthy, J., Büdenbender, J., Czerny, J., Ericson, Y., Esposito, M., Fischer, M., Haunost, M., Hellemann, D., Horn, H. G., Hornick, T., Meyer, J., Sswat, M., Zark, M., and Riebesell, U.: Influence of ocean acidification on a natural winter-to-summer plankton succession: first insights from a long-term mesocosm study draw attention to periods of low nutrient concentrations, PLoS ONE, 11, e0159068, https://doi.org/10.1371/journal.pone.0159068, 2016.
Bacus, S. and Kelley, A.: Effects of ocean acidification and ocean warming on the behavior and physiology of a subarctic, intertidal grazer, Mar. Ecol. Prog. Ser., 711, 31–45, https://doi.org/10.3354/meps14308, 2023.
Bockmon, E. E. and Dickson, A. G.: An inter-laboratory comparison assessing the quality of seawater carbon dioxide measurements, Mar. Chem., 171, 36–43, https://doi.org/10.1016/j.marchem.2015.02.002, 2015.
Bosch, T. C., Krylow, S. M., Bode, H. R., and Steele, R. E.: Thermotolerance and synthesis of heat shock proteins: these responses are present in Hydra attenuata but absent in Hydra oligactis, P. Natl. Acad. Sci. USA, 85, 7927–7931, https://doi.org/10.1073/pnas.85.21.7927, 1988.
Brantley, S. L., Kubicki, J. D., and White, A. F. (Eds.): Kinetics of water-rock interaction, Springer Verlag, New York, 833 pp., https://doi.org/10.1007/978-0-387-73563-4_5, 2008.
Bruno, J. F., Stachowicz, J. J., and Bertness, M. D.: Inclusion of facilitation into ecological theory, Trend. Ecol. Evol., 18, 119–125, https://doi.org/10.1016/S0169-5347(02)00045-9, 2003.
Brzezinski, M. A.: The Si : C : N ratio of marine diatoms: interspecific variability and the effect of some environmental variables, J. Phycol., 21, 347–357, https://doi.org/10.1111/j.0022-3646.1985.00347.x, 1985.
Burt, D. J., Fröb, F., and Ilyina, T.: The sensitivity of the marine carbonate system to regional ocean alkalinity enhancement, Front. Clim., 3, 624075, https://doi.org/10.3389/fclim.2021.624075, 2021.
Byrne, M. and Przeslawski, R.: Multistressor impacts of warming and acidification of the ocean on marine invertebrates' life histories, Integr. Comp. Biol., 53, 582–596, https://doi.org/10.1093/icb/ict049, 2013.
Cailleaud, K., Maillet, G., Budzinski, H., Souissi, S., and Forget-Leray, J.: Effects of salinity and temperature on the expression of enzymatic biomarkers in Eurytemora affinis (Calanoida, Copepoda), Comp. Biochem. Phys. A, 147, 841–849, https://doi.org/10.1016/j.cbpa.2006.09.012, 2007.
Calosi, P., Rastrick, S. P. S., Lombardi, C., De Guzman, H. J., Davidson, L., Jahnke, M., Giangrande, A., Hardege, J. D., Schulze, A., Spicer, J. I., and Gambi, M.-C.: Adaptation and acclimatization to ocean acidification in marine ectotherms: an in situ transplant experiment with polychaetes at a shallow CO2 vent system, Philos. T. Roy. Soc. B, 368, 20120444, https://doi.org/10.1098/rstb.2012.0444, 2013.
Cao, R., Wang, Q., Yang, D., Liu, Y., Ran, W., Qu, Y., Wu, H., Cong, M., Li, F., Ji, C., and Zhao, J.: CO2-induced ocean acidification impairs the immune function of the Pacific oyster against Vibrio splendidus challenge: an integrated study from a cellular and proteomic perspective, Sci. Total Environ., 625, 1574–1583, https://doi.org/10.1016/j.scitotenv.2018.01.056, 2018.
Casadevall, A. and Fang, F. C.: Reproducible science, Infect. Immun., 78, 4972–4975, https://doi.org/10.1128/IAI.00908-10, 2010.
Catlett, D., Matson, P. G., Carlson, C. A., Wilbanks, E. G., Siegel, D. A., and Iglesias-Rodriguez, M. D.: Evaluation of accuracy and precision in an amplicon sequencing workflow for marine protist communities, Limnology & Ocean Methods, 18, 20–40, https://doi.org/10.1002/lom3.10343, 2020.
Clair, T. A. and Hindar, A.: Liming for the mitigation of acid rain effects in freshwaters: a review of recent results, Environ. Rev., 13, 91–128, https://doi.org/10.1139/a05-009, 2005.
Cohen, S., Krueger, T., and Fine, M.: Measuring coral calcification under ocean acidification: methodological considerations for the 45 Ca-uptake and total alkalinity anomaly technique, PeerJ, 5, e3749, https://doi.org/10.7717/peerj.3749, 2017.
Connell, S. D., Kroeker, K. J., Fabricius, K. E., Kline, D. I., and Russell, B. D.: The other ocean acidification problem: CO2 as a resource among competitors for ecosystem dominance, Philos. T. Roy. Soc. B, 368, 20120442, https://doi.org/10.1098/rstb.2012.0442, 2013.
Cornwall, C. E. and Hurd, C. L.: Experimental design in ocean acidification research: problems and solutions, ICES J. Mar. Sci., 73, 572–581, https://doi.org/10.1093/icesjms/fsv118, 2016.
Crain, C. M., Kroeker, K., and Halpern, B. S.: Interactive and cumulative effects of multiple human stressors in marine systems, Ecol. Lett., 11, 1304–1315, https://doi.org/10.1111/j.1461-0248.2008.01253.x, 2008.
Darling, E. S. and Côté, I. M.: Quantifying the evidence for ecological synergies, Ecol. Lett., 11, 1278–1286, https://doi.org/10.1111/j.1461-0248.2008.01243.x, 2008.
Davies, S. P.: Short-term growth measurements of corals using an accurate buoyant weighing technique, Mar. Biol., 101, 389–395, 1989.
DeCarlo, T. M., Comeau, S., Cornwall, C. E., Gajdzik, L., Guagliardo, P., Sadekov, A., Thillainath, E. C., Trotter, J., and McCulloch, M. T.: Investigating marine bio-calcification mechanisms in a changing ocean with in vivo and high-resolution ex vivo Raman spectroscopy, Glob. Change Biol., 25, 1877–1888, https://doi.org/10.1111/gcb.14579, 2019.
De Nooijer, L. J., Van Dijk, I., Toyofuku, T., and Reichart, G. J.: The impacts of seawater Mg Ca and temperature on element incorporation in benthic foraminiferal calcite, Geochem. Geophy. Geosy., 18, 3617–3630, 2017.
Dickson, A. G.: The carbon dioxide system in seawater: equilibrium chemistry and measurements, Guide to best practices for ocean acidification research and data reporting, edited by: Riebesell, U., Fabry, V. J., Hansson, L., and Gattuso, J.-P., Publications Office of the European Union, Luxembourg, https://doi.org/10.2777/66906, ISBN 978-92-79-20650-4, 2010.
Dodge, R. E., Wyers, S. C., Frith, H. R., Knap, A. H., Smith, S. R., Cook, C. B., and Sleeter, T. D.: Coral calcification rates by the buoyant weight technique: effects of alizarin staining, J. Exp. Mar. Biol. Ecol., 75, 217–232, 1984.
Dupont, C. L., Barbeau, K., and Palenik, B.: Ni uptake and limitation in marine Synechococcus strains, Appl. Environ. Microbiol., 74, 23–31, https://doi.org/10.1128/AEM.01007-07, 2008.
Dupont, C. L., Buck, K. N., Palenik, B., and Barbeau, K.: Nickel utilization in phytoplankton assemblages from contrasting oceanic regimes, Deep-Sea Res. Pt. I, 57, 553–566, https://doi.org/10.1016/j.dsr.2009.12.014, 2010.
Esposito, M. C., Boni, R., Cuccaro, A., Tosti, E., and Gallo, A.: Sperm motility impairment in free spawning invertebrates under near-future level of ocean acidification: uncovering the mechanism, Front. Mar. Sci., 6, 794, https://doi.org/10.3389/fmars.2019.00794, 2020.
Farrell, A. P., Eliason, E. J., Sandblom, E., and Clark, T. D.: Fish cardiorespiratory physiology in an era of climate change, Can. J. Zool., 87, 835–851, 2009.
Ferderer, A., Chase, Z., Kennedy, F., Schulz, K. G., and Bach, L. T.: Assessing the influence of ocean alkalinity enhancement on a coastal phytoplankton community, Biogeosciences, 19, 5375–5399, https://doi.org/10.5194/bg-19-5375-2022, 2022.
Figuerola, B., Hancock, A. M., Bax, N., Cummings, V. J., Downey, R., Griffiths, H. J., Smith, J., and Stark, J. S.: A review and meta-analysis of potential impacts of ocean acidification on marine calcifiers from the Southern Ocean, Front. Mar. Sci., 8, 584445, https://doi.org/10.3389/fmars.2021.584445, 2021.
Forbes, V. E. and Calow, P.: Population growth rate as a basis for ecological risk assessment of toxic chemicals, Philos. T. Roy. Soc. Lond. B, 357, 1299–1306, 2002.
Fu, F. X., Zhang, Y., Warner, M. E., Feng, Y., Sun, J., and Hutchins, D. A.: A comparison of future increased CO2 and temperature effects on sympatric Heterosigma akashiwo and Prorocentrum minimum, Harmful Algae, 7, 76–90, https://doi.org/10.1016/j.hal.2007.05.006, 2008.
Fuhr, M., Geilert, S., Schmidt, M., Liebetrau, V., Vogt, C., Ledwig, B., and Wallmann, K.: Kinetics of olivine weathering in seawater: an experimental study, Front. Clim., 4, 831587, https://doi.org/10.3389/fclim.2022.831587, 2022.
Galic, N., Sullivan, L. L., Grimm, V., and Forbes, V. E.: When things don't add up: quantifying impacts of multiple stressors from individual metabolism to ecosystem processing, Ecol. Lett., 21, 568–577, https://doi.org/10.1111/ele.12923, 2018.
Gately, J. A., Kim, S. M., Jin, B., Brzezinski, M. A., and Iglesias-Rodriguez, M. D.: Coccolithophores and diatoms resilient to ocean alkalinity enhancement: a glimpse of hope?, Sci. Adv., 9, eadg6066, https://doi.org/10.1126/sciadv.adg6066, 2023.
Gerhard, M., Koussoroplis, A.-M., Raatz, M., Pansch, C., Fey, S. B., Vajedsamiei, J., Calderó-Pascual, M., Cunillera-Montcusí, D., Juvigny-Khenafou, N. P., and Polazzo, F.: Environmental variability in aquatic ecosystems: avenues for future multifactorial experiments, Limnol. Oceanogr. Lett., 8, 247–266, 2023.
Gilman, S. E., Urban, M. C., Tewksbury, J., Gilchrist, G. W., and Holt, R. D.: A framework for community interactions under climate change, Trend. Ecol. Evol., 25, 325–331, https://doi.org/10.1016/j.tree.2010.03.002, 2010.
Godbold, J. A. and Solan, M.: Long-term effects of warming and ocean acidification are modified by seasonal variation in species responses and environmental conditions, Philos. T. Roy. Soc. B, 368, 20130186, https://doi.org/10.1098/rstb.2013.0186, 2013.
Gradoville, M. R., Bombar, D., Crump, B. C., Letelier, R. M., Zehr, J. P., and White, A. E.: Diversity and activity of nitrogen-fixing communities across ocean basins: diversity and activity of marine nitrogen-fixers, Limnol. Oceanogr., 62, 1895–1909, https://doi.org/10.1002/lno.10542, 2017.
Greatorex, R. and Knights, A. M.: Differential effects of ocean acidification and warming on biological functioning of a predator and prey species may alter future trophic interactions, Mar. Environ. Res., 186, 105903, https://doi.org/10.1016/j.marenvres.2023.105903, 2023.
Gross, M.: Emergency services: a birds eye perspective on the many different functions of stress proteins, Curr. Protein Pept. Sc., 5, 213–223, https://doi.org/10.2174/1389203043379684, 2004.
Guillard, R. R. L. and Ryther, J. H.: Studies of marine planktonic diatoms: i. Cyclotella nana Hustedt, and Detonula confervacea (Cleve) gran., Can. J. Microbiol., 8, 229–239, https://doi.org/10.1139/m62-029, 1962.
Guo, J. A., Strzepek, R., Willis, A., Ferderer, A., and Bach, L. T.: Investigating the effect of nickel concentration on phytoplankton growth to assess potential side-effects of ocean alkalinity enhancement, Biogeosciences, 19, 3683–3697, https://doi.org/10.5194/bg-19-3683-2022, 2022.
Hartmann, J., Suitner, N., Lim, C., Schneider, J., Marín-Samper, L., Arístegui, J., Renforth, P., Taucher, J., and Riebesell, U.: Stability of alkalinity in ocean alkalinity enhancement (OAE) approaches – consequences for durability of CO2 storage, Biogeosciences, 20, 781–802, https://doi.org/10.5194/bg-20-781-2023, 2023.
Harvey, B. P., Gwynn-Jones, D., and Moore, P. J.: Meta-analysis reveals complex marine biological responses to the interactive effects of ocean acidification and warming, Ecol. Evol., 3, 1016–1030, https://doi.org/10.1002/ece3.516, 2013.
Havenhand, J. N., Buttler, F.-R., Thorndyke, M. C., and Williamson, J. E.: Near-future levels of ocean acidification reduce fertilization success in a sea urchin, Curr. Biol., 18, R651–R652, 2008.
Hettinger, A., Sanford, E., Hill, T. M., Russell, A. D., Sato, K. N. S., Hoey, J., Forsch, M., Page, H. N., and Gaylord, B.: Persistent carry-over effects of planktonic exposure to ocean acidification in the Olympia oyster, Ecology, 93, 2758–2768, https://doi.org/10.1890/12-0567.1, 2012.
Hilton, J., Lishman, H., Mackness, S., and Heaney, S. I.: An automated method for the analysis of “particulate” carbon and nitrogen in natural waters, Hydrobiologia, 141, 269–271, 1986.
Ho, T.-Y.: Nickel limitation of nitrogen fixation in Trichodesmium, Limnol. Oceanogr., 58, 112–120, https://doi.org/10.4319/lo.2013.58.1.0112, 2013.
Hu, X.: Effect of organic alkalinity on seawater buffer capacity: a numerical exploration, Aquat. Geochem., 26, 161–178, 2020.
Hurlbert, S. H.: The ancient black art and transdisciplinary extent of pseudoreplication, J. Comp. Psychol., 123, 434–443, https://doi.org/10.1037/a0016221, 2009.
Hutchins, D. A., Fu, F.-X., Zhang, Y., Warner, M. E., Feng, Y., Portune, K., Bernhardt, P. W., and Mulholland, M. R.: CO2 control of Trichodesmium N2 fixation, photosynthesis, growth rates, and elemental ratios: implications for past, present, and future ocean biogeochemistry, Limnol. Oceanogr., 52, 1293–1304, https://doi.org/10.4319/lo.2007.52.4.1293, 2007.
Hutchins, D. A., Fu, F.-X., Yang, S.-C., John, S. G., Romaniello, S. J., Andrews, M. G., and Walworth, N. G.: Responses of globally important phytoplankton groups to olivine dissolution products and implications for carbon dioxide removal via ocean alkalinity enhancement, Microbiology [preprint], https://doi.org/10.1101/2023.04.08.536121, 2023.
Iglesias-Rodriguez, M. D., Halloran, P. R., Rickaby, R. E., Hall, I. R., Colmenero-Hidalgo, E., Gittins, J. R., Green, D. R., Tyrrell, T., Gibbs, S. J., and von Dassow, P.: Phytoplankton calcification in a high-CO2 world, Science, 320, 336–340, 2008.
Johnson D. W.: Selection on offspring size and contemporary evolution under ocean acidification, Nat. Clim. Change 12, 757–760, 2022.
Kelly, M. W., Padilla-Gamiño, J. L., and Hofmann, G. E.: Natural variation and the capacity to adapt to ocean acidification in the keystone sea urchin Strongylocentrotus purpuratus, Glob. Change Biol., 19, 2536–2546, 2013.
Khalil, M., Doo, S. S., Stuhr, M., and Westphal, H.: Long term physiological responses to combined ocean acidifcation and warming show energetic trade ofs in an asterinid starfish, Coral Reefs, 42, 845–858, https://doi.org/10.1007/s00338-023-02388-2, 2023.
Kim, H.-C. and Lee, K.: Significant contribution of dissolved organic matter to seawater alkalinity, Geophys. Res. Lett., 36, L20603, https://doi.org/10.1029/2009GL040271, 2009.
Koeve, W., Kim, H.-C. Lee, K., and Oschlies, A: Computation of fCO2 and the concentration of carbonate ions and the potential role of DOM accumulation in ocean acidification experiments, Joint EPOCA, BIOACID an UKOARP Meeting, 27–30 September 2010, Atlantic Hotel, Bremerhaven, Germany, p. 30, https://oceanrep.geomar.de/id/eprint/11788/ (last access: 16 November 2023), 2010.
Kroeker, K. J. and Sanford, E.: Ecological leverage points: species interactions amplify the physiological effects of global environmental change in the ocean, Annu. Rev. Mar. Sci., 14, 75–103, https://doi.org/10.1146/annurev-marine-042021-051211, 2022.
Kroeker, K. J., Kordas, R. L., Crim, R. N., and Singh, G. G.: Meta-analysis reveals negative yet variable effects of ocean acidification on marine organisms: biological responses to ocean acidification, Ecol. Lett., 13, 1419–1434, https://doi.org/10.1111/j.1461-0248.2010.01518.x, 2010.
Kroeker, K. J., Kordas, R. L., Crim, R., Hendriks, I. E., Ramajo, L., Singh, G. S., Duarte, C. M., and Gattuso, J.: Impacts of ocean acidification on marine organisms: quantifying sensitivities and interaction with warming, Glob. Change Biol., 19, 1884–1896, https://doi.org/10.1111/gcb.12179, 2013a.
Kroeker, K. J., Micheli, F., and Gambi, M. C.: Ocean acidification causes ecosystem shifts via altered competitive interactions, Nat. Clim. Change, 3, 156–159, https://doi.org/10.1038/nclimate1680, 2013b.
LaRoche, J., Rost, B., and Engel, A.: Bioassays, batch culture and chemostat experimentation, in: Approaches and tools to manipulate the carbonate chemistry, Guide for Best Practices in Ocean Acidification Research and Data Reporting, edited by: Riebesell, U., Fabry, V. J., Hansson, L., and Gattuso, J.-P., 81–94, https://doi.org/10.2777/66906, ISBN 978-92-79-20650-4, 2010.
La Terza, A., Papa, G., Miceli, C., and Luporini, P.: Divergence between two Antarctic species of the ciliate Euplotes, E. focardii and E. nobilii, in the expression of heat-shock protein 70 genes, Mol. Ecol., 10, 1061–1067, https://doi.org/10.1046/j.1365-294X.2001.01242.x, 2001.
Lawrence, M. J., Eliason, E. J., Brownscombe, J. W., Gilmour, K. M., Mandelman, J. W., and Cooke, S. J.: An experimental evaluation of the role of the stress axis in mediating predator-prey interactions in wild marine fish, Comp. Biochem. Phys. A, 207, 21–29, 2017.
Lee, Y. H., Kim, M.-S., Wang, M., Bhandari, R. K., Park, H. G., Wu, R. S.-S., and Lee, J.-S.: Epigenetic plasticity enables copepods to cope with ocean acidification, Nat. Clim. Change, 12, 918–927, 2022.
Lesser, M. P.: Oxidative stress in marine environments: biochemistry and physiological ecology, Annu. Rev. Physiol., 68, 253–278, https://doi.org/10.1146/annurev.physiol.68.040104.110001, 2006.
Li, Y., Liew, Y. J., Cui, G., Cziesielski, M. J., Zahran, N., Michell, C. T., Voolstra, C. R., and Aranda, M.: DNA methylation regulates transcriptional homeostasis of algal endosymbiosis in the coral model Aiptasia, Sci. Adv., 4, eaat2142, https://doi.org/10.1126/sciadv.aat2142, 2018.
Liu, G., Innes, D., and Thompson, R. J.: Quantitative analysis of sperm plane circular movement in the blue mussels Mytilus edulis, M. trossulus and their hybrids, J. Exp. Zool. Part A, 315, 280–290, 2011.
Lushchak, V. I.: Environmentally induced oxidative stress in aquatic animals, Aquat. Toxicol., 101, 13–30, https://doi.org/10.1016/j.aquatox.2010.10.006, 2011.
Ma, K. C. K., Monsinjon, J. R., Froneman, P. W., and McQuaid, C. D.: Thermal stress gradient causes increasingly negative effects towards the range limit of an invasive mussel, Sci. Total Environ., 865, 161184, https://doi.org/10.1016/j.scitotenv.2022.161184, 2023.
Maranhão, P. and Marques, J. C.: The influence of temperature and salinity on the duration of embryonic development, fecundity and growth of the amphipod Echinogammarus marinus Leach (Gammaridae), Acta Oecol., 24, 5–13, 2003.
Maranón, E., Cermeno, P., Fernández, E., Rodríguez, J., and Zabala, L.: Significance and mechanisms of photosynthetic production of dissolved organic carbon in a coastal eutrophic ecosystem, Limnol. Oceanogr., 49, 1652–1666, 2004.
Martin, B., Jager, T., Nisbet, R. M., Preuss, T. G., and Grimm, V.: Limitations of extrapolating toxic effects on reproduction to the population level, Ecol. Appl., 24, 1972–1983, 2014.
Martz, T. R., Daly, K. L., Byrne, R. H., Stillman, J. H., and Turk, D.: Technology for ocean acidification research: needs and availability, Oceanography, 28, 40–47, 2015.
Masson-Delmotte, V., Zhai, P., Pirani, A., Connors, S. L., Péan, C., Berger, S., Caud, N., Chen, Y., Goldfarb, L., and Gomis, M. I.: Climate change 2021: the physical science basis, Contribution of working group I to the sixth assessment report of the intergovernmental panel on climate change, Cambridge University Press, Cambridge, United Kingdom and New York, NY, USA, https://doi.org/10.1017/9781009157896, 2021.
Matsumoto, K., Tanioka, T., and Rickaby, R.: Linkages between dynamic phytoplankton C : N : P and the ocean carbon cycle under climate change, Oceanography, 33, 44–52, 2020.
Middelburg, J. J., Soetaert, K., and Hagens, M.: Ocean alkalinity, buffering and biogeochemical processes, Rev. Geophys., 58, e2019RG000681, https://doi.org/10.1029/2019RG000681, 2020.
Millero, F. J., Woosley, R., DiTrolio, B., and Waters, J.: Effect of ocean acidification on the speciation of metals in seawater, Oceanography, 22, 72–85, 2009.
Mitchell, A., Hayes, C., Booth, D. J., and Nagelkerken, I.: Future shock: ocean acidification and seasonal water temperatures alter the physiology of competing temperate and coral reef fishes, Sci. Total Environ., 883, 163684, https://doi.org/10.1016/j.scitotenv.2023.163684, 2023.
Montoya, J. P., Voss, M., Kahler, P., and Capone, D. G.: A simple, high-precision, high-sensitivity tracer assay for N(inf2) fixation, Appl. Environ. Microbiol., 62, 986–993, https://doi.org/10.1128/aem.62.3.986-993.1996, 1996.
Montserrat, F., Renforth, P., Hartmann, J., Leermakers, M., Knops, P., and Meysman, F. J. R.: Olivine dissolution in seawater: implications for CO2 sequestration through enhanced weathering in coastal environments, Environ. Sci. Technol., 51, 3960–3972, https://doi.org/10.1021/acs.est.6b05942, 2017.
Moras, C. A., Bach, L. T., Cyronak, T., Joannes-Boyau, R., and Schulz, K. G.: Ocean alkalinity enhancement – avoiding runaway CaCO3 precipitation during quick and hydrated lime dissolution, Biogeosciences, 19, 3537–3557, https://doi.org/10.5194/bg-19-3537-2022, 2022.
Morel, F. M., Rueter, J. G., Anderson, D. M., and Guillard, R. R. L.: Aquil: a chemically defined phytoplankton culture medium for trace metal studies, J. Phycol., 15, 135–141, 1979.
Moya, A., Huisman, L., Foret, S., Gattuso, J.-P., Hayward, D. C., Ball, E. E., and Miller, D. J.: Rapid acclimation of juvenile corals to CO2-mediated acidification by upregulation of heat shock protein and Bcl-2 genes, Mol. Ecol., 24, 438–452, 2015.
National Academies of Sciences, Engineering, and Medicine (NASEM): A research strategy for ocean-based carbon dioxide removal and sequestration, National Academies Press, Washington, D.C., https://doi.org/10.17226/26278, 2022.
Nawaz, S., Lezaun, J., Valenzuela, J. M., and Renforth, P.: Broaden research on ocean alkalinity enhancement to better characterize social impacts, Environ. Sci. Technol., 57, 8863–8869, https://doi.org/10.1021/acs.est.2c09595, 2023.
Nielsen, E. S.: The use of radio-active carbon (C14) for measuring organic production in the sea, ICES J. Mar. Sci., 18, 117–140, 1952.
O'Donnell, M. J., Hammond, L. M., and Hofmann, G. E.: Predicted impact of ocean acidification on a marine invertebrate: elevated CO2 alters response to thermal stress in sea urchin larvae, Mar. Biol., 156, 439–446, 2009.
Paiva, F., Brennecke, D., Pansch, C., and Briski, E.: Consistency of aquatic enclosed experiments: the importance of scale and ecological complexity, Divers. Distrib., 27, 524–532, 2021.
Paul, A. J., Bach, L. T., Schulz, K.-G., Boxhammer, T., Czerny, J., Achterberg, E. P., Hellemann, D., Trense, Y., Nausch, M., Sswat, M., and Riebesell, U.: Effect of elevated CO2 on organic matter pools and fluxes in a summer Baltic Sea plankton community, Biogeosciences, 12, 6181–6203, https://doi.org/10.5194/bg-12-6181-2015, 2015.
Paul, A. J., Achterberg, E. P., Bach, L. T., Boxhammer, T., Czerny, J., Haunost, M., Schulz, K.-G., Stuhr, A., and Riebesell, U.: No observed effect of ocean acidification on nitrogen biogeochemistry in a summer Baltic Sea plankton community, Biogeosciences, 13, 3901–3913, https://doi.org/10.5194/bg-13-3901-2016, 2016.
Pistevos, J. C. A., Nagelkerken, I., Rossi, T., and Connell, S. D.: Antagonistic effects of ocean acidification and warming on hunting sharks, Oikos, 126, oik.03182, https://doi.org/10.1111/oik.03182, 2017.
Place, S. P. and Hofmann, G. E.: Constitutive expression of a stress-inducible heat shock protein gene, hsp70, in phylogenetically distant Antarctic fish, Polar Biol., 28, 261–267, https://doi.org/10.1007/s00300-004-0697-y, 2005.
Pujo-Pay, M. and Raimbault, P.: Improvement of the wet-oxidation procedure for simultaneous determination of particulate organic nitrogen and phosphorus collected on filters, Mar. Ecol. Prog. Ser., 105, 203–203, 1994.
Ragg, N. L., Gale, S. L., Le, D. V., Hawes, N. A., Burritt, D. J., Young, T., Ericson, J. A., Hilton, Z., Watts, E., and Berry, J.: The effects of aragonite saturation state on hatchery-reared larvae of the Greenshell mussel Perna canaliculus, J. Shellfish Res., 38, 779–793, 2019.
Renforth, P. and Henderson, G.: Assessing ocean alkalinity for carbon sequestration, Rev. Geophys., 55, 636–674, 2017.
Reusch, T. B.: Climate change in the oceans: evolutionary versus phenotypically plastic responses of marine animals and plants, Evol. Appl., 7, 104–122, 2014.
Ridgwell, A., Schmidt, D. N., Turley, C., Brownlee, C., Maldonado, M. T., Tortell, P., and Young, J. R.: From laboratory manipulations to Earth system models: scaling calcification impacts of ocean acidification, Biogeosciences, 6, 2611–2623, https://doi.org/10.5194/bg-6-2611-2009, 2009.
Ries, J., Ghazaleh, M., Connolly, B., Westfield, I., and Castillo, K.: Impacts of ocean acidification and warming on the dissolution kinetics of whole-shell biogenic carbonates, Geochim. Cosmochim. Ac., 192, 318–337, 2016.
Ries, J. B.: Effect of ambient Mg Ca ratio on Mg fractionation in calcareous marine invertebrates: a record of the oceanic Mg Ca ratio over the Phanerozoic, Geology, 32, 981–984, 2004.
Ries, J. B.: Mg fractionation in crustose coralline algae: geochemical, biological, and sedimentological implications of secular variation in the Mg Ca ratio of seawater, Geochim. Cosmochim. Ac., 70, 891–900, 2006.
Ries, J. B.: Review: geological and experimental evidence for secular variation in seawater Mg Ca (calcite-aragonite seas) and its effects on marine biological calcification, Biogeosciences, 7, 2795–2849, https://doi.org/10.5194/bg-7-2795-2010, 2010.
Ries, J. B., Cohen, A. L., and McCorkle, D. C.: Marine calcifiers exhibit mixed responses to CO2-induced ocean acidification, Geology, 37, 1131–1134, 2009.
Saigusa, M.: Control of hatching in an estuarine terrestrial crab I. Hatching of embryos detached from the female and emergence of mature larvae, Biol. Bull., 183, 401–408, 1992.
Sala, M. M., Aparicio, F. L., Balagué, V., Boras, J. A., Borrull, E., Cardelús, C., Cros, L., Gomes, A., López-Sanz, A., Malits, A., Martínez, R. A., Mestre, M., Movilla, J., Sarmento, H., Vázquez-Domínguez, E., Vaqué, D., Pinhassi, J., Calbet, A., Calvo, E., Gasol, J. M., Pelejero, C., and Marrasé, C.: Contrasting effects of ocean acidification on the microbial food web under different trophic conditions, ICES J. Mar. Sci., 73, 670–679, https://doi.org/10.1093/icesjms/fsv130, 2016.
Sanders, T., Schmittmann, L., Nascimento-Schulze, J. C., and Melzner, F.: High calcification costs limit mussel growth at low salinity, Front. Mar. Sci., 5, 352, https://doi.org/10.3389/fmars.2018.00352, 2018.
Schulte, P. M.: What is environmental stress? Insights from fish living in a variable environment, J. Exp. Biol., 217, 23–34, https://doi.org/10.1242/jeb.089722, 2014.
Sharp, J. H., Benner, R., Bennett, L., Carlson, C. A., Fitzwater, S. E., Peltzer, E. T., and Tupas, L. M.: Analyses of dissolved organic carbon in seawater: the JGOFS EqPac methods comparison, Mar. Chem., 48, 91–108, 1995.
Stachowicz, J. J.: Mutualism, facilitation, and the structure of ecological communities, BioScience, 51, 235–246, https://doi.org/10.1641/0006-3568(2001)051[0235:MFATSO]2.0.CO;2, 2001.
Subhas, A. V., Marx, L., Reynolds, S., Flohr, A., Mawji, E. W., Brown, P. J., and Cael, B. B.: Microbial ecosystem responses to alkalinity enhancement in the North Atlantic Subtropical Gyre, Front. Clim., 4, 784997, https://doi.org/10.3389/fclim.2022.784997, 2022.
Thor, P. and Dupont, S.: Transgenerational effects alleviate severe fecundity loss during ocean acidification in a ubiquitous planktonic copepod, Glob. Change Biol., 21, 2261–2271, 2015.
Trimborn, S., Thoms, S., Brenneis, T., Heiden, J. P., Beszteri, S., and Bischof, K.: Two Southern Ocean diatoms are more sensitive to ocean acidification and changes in irradiance than the prymnesiophyte Phaeocystis antarctica, Physiol. Plantarum, 160, 155–170, 2017.
Vandamme, D., Pohl, P. I., Beuckels, A., Foubert, I., Brady, P. V., Hewson, J. C., and Muylaert, K.: Alkaline flocculation of Phaeodactylum tricornutum induced by brucite and calcite, Bioresource Technol., 196, 656–661, 2015.
Vehmaa, A., Hogfors, H., Gorokhova, E., Brutemark, A., Holmborn, T., and Engström-Öst, J.: Projected marine climate change: effects on copepod oxidative status and reproduction, Ecol. Evol., 3, 4548–4557, https://doi.org/10.1002/ece3.839, 2013.
Verardo, D. J., Froelich, P. N., and McIntyre, A.: Determination of organic carbon and nitrogen in marine sediments using the Carlo Erba NA-1500 Analyzer, Deep-Sea Res. Pt. A, 37, 157–165, 1990.
Voigt, W., Perner, J., Davis, A. J., Eggers, T., Schumacher, J., Bährmann, R., Fabian, B., Heinrich, W., Köhler, G., Lichter, D., Marstaller, R., and Sander, F. W.: Trophic levels are differentially sensitive to climate, Ecology, 84, 2444–2453, https://doi.org/10.1890/02-0266, 2003.
Von Weissenberg, E., Jansson, A., Vuori, K. A., and Engström-Öst, J.: Copepod reproductive effort and oxidative status as responses to warming in the marine environment, Ecol. Evol., 12, e8594, https://doi.org/10.1002/ece3.8594, 2022.
Wang, H., Pilcher, D. J., Kearney, K. A., Cross, J. N., Shugart, O. M., Eisaman, M. D., and Carter, B. R.: Simulated impact of ocean alkalinity enhancement on atmospheric CO2 removal in the Bering Sea, Earth's Future, 11, e2022EF002816, https://doi.org/10.1029/2022EF002816, 2023.
Wernberg, T., Smale, D. A., and Thomsen, M. S.: A decade of climate change experiments on marine organisms: procedures, patterns and problems, Glob. Change Biol., 18, 1491–1498, https://doi.org/10.1111/j.1365-2486.2012.02656.x, 2012.
White, A. E., Granger, J., Selden, C., Gradoville, M. R., Potts, L., Bourbonnais, A., Fulweiler, R. W., Knapp, A. N., Mohr, W., Moisander, P. H., Tobias, C. R., Caffin, M., Wilson, S. T., Benavides, M., Bonnet, S., Mulholland, M. R., and Chang, B. X.: A critical review of the 15N2 tracer method to measure diazotrophic production in pelagic ecosystems, Limnol. Oceanogr. Meth., 18, 129–147, https://doi.org/10.1002/lom3.10353, 2020.
Worsfold, P. J., Clough, R., Lohan, M. C., Monbet, P., Ellis, P. S., Quétel, C. R., Floor, G. H., and McKelvie, I. D.: Flow injection analysis as a tool for enhancing oceanographic nutrient measurements – a review, Anal. Chim. Acta, 803, 15–40, 2013.
Wuebbles, D. J., Fahey, D. W., Hibbard, K. A., Dokken, D. J., Stewart, B. C., and Maycock, T. K. (Eds.): USGCRP: Climate Science Special Report: Fourth National Climate Assessment, Volume I, U.S. Global Change Research Program, Washington, DC, USA, 470 pp., https://doi.org/10.7930/J0J964J6, 2017.
Yang, B., Leonard, J., and Langdon, C.: Seawater alkalinity enhancement with magnesium hydroxide and its implication for carbon dioxide removal, Mar. Chem., 253, 104251, https://doi.org/10.1016/j.marchem.2023.104251, 2023.
- Abstract
- Introduction
- Lessons learned from ocean acidification research
- Seawater media preparation and manipulation of carbonate chemistry
- Experimental design
- Sampling and analysis
- Conclusions and recommendations
- Data availability
- Author contributions
- Competing interests
- Disclaimer
- Acknowledgements
- Financial support
- Review statement
- References
- Abstract
- Introduction
- Lessons learned from ocean acidification research
- Seawater media preparation and manipulation of carbonate chemistry
- Experimental design
- Sampling and analysis
- Conclusions and recommendations
- Data availability
- Author contributions
- Competing interests
- Disclaimer
- Acknowledgements
- Financial support
- Review statement
- References