the Creative Commons Attribution 4.0 License.
the Creative Commons Attribution 4.0 License.
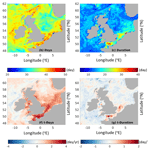
Characteristics and trends of marine heatwaves in the northwest European Shelf and the impacts on density stratification
Joanna Staneva
Marine heatwaves (MHWs) are characterized by anomalous and prolonged increases in sea surface temperatures driven by atmospheric and oceanic factors. The intensification of MHWs is an evident consequence of ongoing global climate change. The question of whether the northwest European Shelf (NWES) is experiencing increased stratification in recent decades is of significant interest with respect to understanding the impacts of these extreme events. In this study, we leverage ocean physics reanalysis data obtained from the Copernicus Marine Environment Monitoring Service (CMEMS) covering the temporal span from 1993 to 2023 to conduct a rigorous examination of the NWES domain. The focus centers on the assessment of potential energy anomaly (PEA) and its role in shaping stratification dynamics.
Our findings reveal an increase in both the frequency and duration of MHWs in the NWES area, especially in coastal areas, where the duration of MHWs is increasing the fastest, generally by more than 2 d yr−1 over the study period. However, despite the intensified MHWs, thermal stratification in the NWES is weakening, particularly in the middle and northern North Sea. This suggests that the warming effect due to MHWs is insufficient to counteract the overall decline in thermal stratification caused by global warming. Additionally, our study highlights the significance of seawater salinity in driving the trend of density stratification. Specifically, the discharge from the Baltic Sea plays a crucial role in influencing the stratification patterns in the North Sea region.
- Article
(4470 KB) - Full-text XML
- BibTeX
- EndNote
Marine heatwaves (MHWs) are extreme oceanic events characterized by unusually warm sea surface temperatures (SSTs) that exceed the local 90th percentile for at least 5 consecutive days (Hobday et al., 2016). These events are characterized by their intensity, duration, and spatial extent, often leading to ecological disturbances and significant shifts in species distribution patterns (Frölicher et al., 2018). MHWs can occur in various oceanic regions, including coastal areas, and the occurrence of MHW events has shown an increasing trend globally over the past century (Oliver et al., 2018; IPCC, 2021).
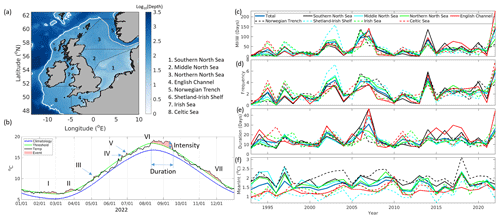
Figure 1(a) Map of the northwest European Shelf Sea with sub-regional division (data from Table 1, ref. 1). Dashed curve indicates 200 m isobath. (b) Detection of MHW events and their characteristics in 2022 (data from Table 1, ref. 1) near the Dogger Bank region in the southern North Sea (region 1 in panel (a)). (c–f) Variations in the MHW characteristics between 1993 and 2022, with the bold solid curve indicating the mean of total subdomains of the NWES (daily SST data from Table 1, ref. 1).
Climate models project a continued upward trend in the occurrence of MHWs in the coming decades that is driven by anthropogenic climate change (Frölicher et al., 2018; Oliver et al., 2019, 2020; IPCC, 2021). The northwest European Shelf (NWES), which is a large area of shallow temperate sea located between 47–61° N latitude and 12° W–10° E (Fig. 1), is expected to experience a similar trend of increasing MHW events (IPCC, 2021). Understanding the dynamics of MHWs and their consequences is crucial for effective ecosystem management and conservation efforts (Smale et al., 2019).
Elevated SSTs can lead to widespread and severe ecological disturbances, including shifts in species distributions, alterations in community structure, and increased vulnerability to invasive species (Oliver et al., 2018; Smale et al., 2019). These events can disrupt important ecological processes, such as nutrient cycling, primary production, and trophic interactions, with cascading effects on the entire marine food web (Wernberg et al., 2013, 2016; Oliver et al., 2020). In the NWES region, the increasing frequency of MHW occurrence is anticipated to have significant consequences for both the socioeconomic system and natural processes. For instance, Borges et al. (2019) observed a 3-fold increase in dissolved methane concentration in surface waters along the Belgian coast during the summer of 2018 compared to a typical year. Additionally, MHWs have been implicated in the occurrence and persistence of thermal stratification, leading to changes in vertical mixing and nutrient availability in the water column (Chen et al., 2022). Such alterations in thermal stratification can have profound implications for the functioning of marine ecosystems and their resilience to climate change (Herring et al., 2015). The recently published Copernicus Marine Service Ocean State Report, Issue 5 (Wakelin et al., 2021), documented the identification of extreme temperature events and their potential impacts on important fish and shellfish stocks. However, a lack of systematic studies to elucidate the long-term relationship between the vertical stratification and MHWs hampers our understanding of the impacts of extreme temperature events on ecosystem stability.
Given the potential consequences of increased MHW frequency on the NWES region, it is essential to address the following research question: is the NWES becoming more stratified due to the increased frequency of marine heatwave occurrence? Addressing this question requires a comprehensive assessment of long-term observational data, climate model simulations, and advanced analytical techniques to examine the relationships between MHW events, thermal stratification, and their ecological implications. This research will provide valuable insights into the potential impacts of MHWs on the NWES ecosystem and contribute to our understanding of the broader effects of climate change on marine environments.
The three-dimensional water temperature and salinity data from the Copernicus Marine Environment Monitoring Service (CMEMS) ocean physics reanalysis data (Table 1, product ref. 1) is applied in this study. These data cover the NWES with the assimilation model at 7 km horizontal resolution for the period 1993 to 2022. The modeled temperature and salinity are validated through comparisons with in situ observations from the World Ocean Database, mooring data, and the multimodel ensemble of multiyear products, which is an internal CMEMS product. More details of the CMEMS products are given in Table 1.
Moreover, the CMEMS SST reanalysis is extended by the European Space Agency Sea Surface Temperature Climate Change Initiative (ESA SST CCI; Table 1, product ref. 1) Level 3 product for the period 1982–1992 (Merchant et al., 2019). This product has a spatial resolution of 0.05° by 0.05° for the northwest Atlantic Shelf region. The ESA dataset is also employed as observational data for assimilating CMEMS data (see the quality information document (QUID) and product user manual (PUM) of the product; Table 1). All SST data are interpolated on the same spatial grid as the CMEMS product ref. 1 (Table 1), such that the 40-year period provides the baseline climatology reference period for computing the seasonally varying 90th percentile threshold, as defined in Hobday et al. (2016). The MATLAB toolbox by Zhao and Marin (2019) is applied for detecting MHW events and to properly computing means and trends of MHW properties.
The potential energy anomaly is used as a measure of the degree of density stratification as follows (Simpson, 1981):
in which
is the vertical mean water density, and g = 9.8 m s−2 is the gravitational acceleration. The instantaneous total water depth is given by , with η and H being the sea surface elevation and the time mean water depth, respectively. The potential energy anomaly measures the amount of mechanical energy (per m3) required to instantaneously homogenize the water column with given density stratification. The water density ρ was calculated (at 1 atm), following Millero and Poisso (1981):
In Eq. (3), S is the salinity of seawater in pptv (parts per thousand by volume). The reference density ρr and the coefficients A, B, and C are also functions of temperature T in degrees Celsius, with expressions given by Millero and Poisso (1981):
Here, S and T, as well as the sea surface elevation η and water depth H (see Eqs. 1 and 2), are obtained from the CMEMS products.
Furthermore, the sensitivity of density stratification to the occurrence of MHWs is quantified with the ratio between the varying heatwave days and varying water-stratified days (Chen et al., 2022):
Here, the number of MHW days (M) and the number of days that the water column was stratified (N) are counted in each year (i). The parameter n=30 (i.e., 1993–2022) indicates the length of the computing period. The overline denotes the multiyear mean.
A MHW in each year is characterized by the number of events and the duration and intensity of each event. As an example, Fig. 1b illustrates the detection of MHW events near the Dogger Bank region (region 1 in Fig. 1a), in the middle of the North Sea in 2022. The MHW occurs multiple times throughout the whole year, even during winter. The duration of each event varies from 5 d (e.g., events 4 and 5) to 40 d (events 6 and 7). The intensity, which measures the deviation of SST from the threshold, reaches its maximum of 2 °C in late September.
MHWs are exceptionally active in the year 2022. The total number of days of MHWs reaches 140 d on average (Fig. 1c), with the maximum exceeding 200 d in the English Channel. The lowest number of MHW days, 60 d, is found in the Norwegian Trench. Throughout this year, the Celtic Sea experienced more than seven MHW events. Apart from the events in 2022, MHWs were also active in the years 1995, 1997, 2003, 2007, and 2014, during which three or more MHW events were observed (Fig. 1d).
The occurrence of MHWs undergoes large temporal variations between the years 1993 and 2022, when MHW days appear much longer than during other years. During 1993–2022, the general duration of a MHW event is around 10 to 20 d (Fig. 1e). Longer durations are also observed. For example, in 2007, a duration with more than 40 d duration was observed in the English Channel and the Celtic Sea (region 8; Fig. 1a). The annual mean intensity of MHWs ranges on average between 1 and 2 °C and shows insignificant differences with the occurrences of MHW, the number of days, or the length of the duration (Fig. 1f). However, regional dependence can be observed. For example, in the Shetland–Irish Shelf (region 6; Fig. 1a), the mean intensity varies between 1 and 1.5 °C over the period 1993 to 2022. It also becomes stronger in the Irish Sea (region 7; Fig. 1a) and the North Sea (regions 1–3; Fig. 1a) over these periods. In general, the annual mean intensity of MHWs occurring in the Norwegian Trench (region 4; Fig. 1a) can reach values of up to 2 to 3 °C (Fig. 1f), which is more intense than in the other regions.
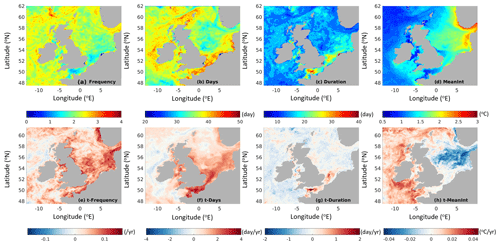
Figure 2Mean and trend values of MHW over the past 30 years (1993–2022). Dashed lines indicate 200 m isobath (daily SST data from Table 1, ref. 1).
The mean and trend values of MHWs over the past 30 years are shown in Fig. 2. The lowest frequency of the occurrence of MHWs is found at the southern North Sea and the English Channel, where on average only one to two MHW events occurred every year (Fig. 2a). The Shetland–Irish Shelf and the Celtic Sea experienced two to three MHW events. Concurrently, all these regions had longer MHW periods (approximately 40–50 d) than the middle and northern North Sea (Fig. 2b). Furthermore, it is found that MHW events last longer further to the south. The longest duration, 30–40 d, occurred near the coast of the North Sea and the English Channel (Fig. 2c). This indicates that the MHWs that appear in the southern part of the NWES are mostly continuous and long term, while the MHWs that appear in the northern part of the NWES and the shelf edge are mostly intermittent and short term.
The occurrence of MHWs can be primarily attributed to the following two drivers: local air–sea heat exchange resulting from abnormally high air temperatures and nonlocal heat transport via ocean advection (Gupta et al., 2020; Schlegel et al., 2021). The atmospheric factor emerges as the predominant driver of MHWs in the southern to middle North Sea (Chen et al., 2022; Mohamed et al., 2023). Nonlocal heat fluxes, such as the influx of warm Atlantic water into NWES, may be responsible for the development of MHWs.
Compared to the long-term average, higher seawater temperatures will result in more heat fluxes into the NWES by the North Atlantic shelf current, particularly in the English Channel and the Shetland–Irish Shelf (zones 4 and 6 in Fig. 1a). The heightened seawater levels make these areas more prone to experiencing MHWs compared to regions less affected by the North Atlantic current, such as the Norwegian Trench (zone 5; Fig. 1a). This may explain why these regions have more days with MHWs. Furthermore, the mean intensity of MHWs in these two regions is notably lower than in the Norwegian Trench (Fig. 2d), which supports the assertion. The lowest mean intensity is observed in the Irish Sea and the English Channel. The MHW intensifies towards the east coast of the NWES. Along the coast of Denmark and Norway, the mean intensity reaches approximately 2.5–3 °C. However, compared to the southern NWES, the shorter durations and higher frequencies of MHWs in its northern region may be attributed to the distinct characteristics of climate drivers in their respective areas. This is because atmospheric influences, in contrast to oceanic influences, exhibit larger variability in affecting SST (Tinker and Howes, 2020). Other drivers, such as local wind (Mohamed et al., 2023), may introduce further uncertainties to the occurrence and persistence of MHWs. Identifying the dominant drivers of MHW features in NWES requires a systematic investigation of the relationship between air and sea temperature in various regions. However, this detailed analysis is not elaborated on in this paper due to space constraints.
Over the past 3 decades, the frequency of MHWs increased at a rate of 0.1–0.15 yr−1 (Fig. 2e). Correspondingly, the number of days experiencing MHWs increased by 1 to 4 d yr−1 (Fig. 2f). Coastal areas are where the number of days increases the fastest, with generally more than 2 d yr−1 over the study period. The fastest-increasing region is the English Channel, reaching or even exceeding 4 d yr−1. The duration of the MHW events shows no significant trend in the NWES, except for the English Channel, where each MHW event was 1 to 2 d longer, corresponding to increasing MHW days (Fig. 2g). The mean intensity (Fig. 2h) shows a completely different trend in the North Sea and the rest of the regions. In the North Sea, MHWs tend to be less intense, whereas in the Shetland–Irish Shelf and the Celtic Sea, MHWs intensified at a rate of 0.02–0.04 °C yr−1. In the English Channel and the western part of the southern North Sea, the annual mean intensity remained the same from 1993 to 2023.
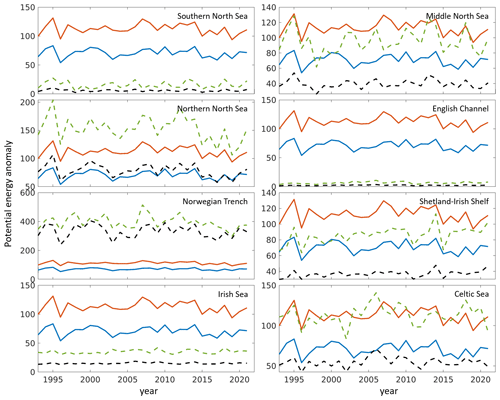
Figure 3Potential energy anomaly (∅; J m−3) between 1993 and 2022. The solid curves denote the spatial mean PEA of the entire NWES domain. The red curves represent the summer period (June–September) mean in red, and the blue curves show the annual mean. The spatial mean PEA of different subdomains of the NWES (see Fig. 1a) is indicated by dashed curves, with green and black for the annual mean and summer mean, respectively.
The degree of density stratification, quantified by the potential energy anomaly (PEA) ∅, is shown in Fig. 3. In this study, only the annual mean and summer period (June to September) mean stratification are considered. Following Chen et al. (2022), the water column is considered stratified when ∅ ≥ 50 J m−3. The NWES is weakly stratified with ∅ ≈ 70 J m−3. During summer, higher SST enhances the density stratification, leading to ∅ in summer being approximately twice as high as the annual mean. The PEA is low in the southern part of the North Sea, the Irish Sea, where the depth is shallower. Due to strong tides, the water column is generally well mixed in the English Channel (Pohlmann, 1996). In the middle North Sea and the Shetland–Irish Shelf, ∅ ≥ 50 J m−3 during the summertime and ∅ < 50 J m−3 for the annual mean presents an obvious seasonal summer stratification. The Celtic Sea also exhibits seasonal cycles in PEA, with ∅ being 110–120 J m−3 during the summer and around 50 J m−3 over the entire year. The large annual mean PEA is mainly attributed to the extended warming period and stratification during autumn. The northern North Sea shows a seasonal cycle similar to that of the middle North Sea but with a larger ∅, both in the summer period and in the annual mean, due to larger depth. The potential energy anomaly in the Norwegian Trench is ∼ 400 J m−3. This is much larger than what is observed for the NWES region as a whole.
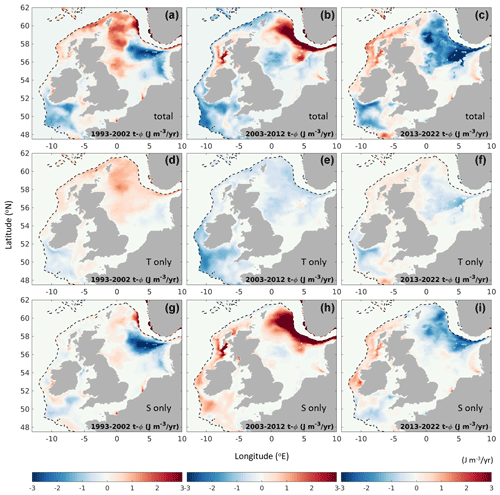
Figure 4Trend of potential energy anomaly (∅; J m−3 yr−1) over the last 30 years. The first row (total) shows ∅ computed with Eq. (1), where density depends on both temperature (T) and salinity (S) in the water column. The second and third rows are similar to the first row, but the density depends only on either T or S, respectively.
The density stratification has exhibited a trend opposite to that of MHWs over the past 30 years. Figure 4 illustrates the 10-year-averaged PEA across 3 decades, spanning from 1993 to 2022. Evident shifts in stratification are discernible. An obvious transformation of stratification is observed. Between 1993 and 2022, there was a decrease at a rate of −1 to −2 J m−3 yr−1 only in the eastern part of the southern and middle North Sea, as well as in the Celtic Sea. Conversely, in the eastern part of the middle North Sea, the northern North Sea, and the Shetland–Irish Shelf, ∅ increased by approximately 1–1.5 J m−3 yr−1. During the second decade, the region where stratification grows was greatly reduced. Only parts of the northern North Sea near the Norwegian Trench and some areas of the Shetland–Irish Shelf still maintained a growth rate of 1 to 2 J m−3 yr−1. In the third decade, the entire North Sea tended to be less stratified, especially in the middle and northern parts of the North Sea, where the potential energy anomaly decreased by −2 to −3 J m−3 yr−1. The transformation in the Norwegian Trench is consistent with that in the NWES (i.e., from an increased stratification from 1993 to 2012 to a decreased stratification in the most recent decade), although at a relatively higher rate (6–8 J m−3 yr−1).
The PEA trend is further decomposed to that due to the seawater temperature (T only; Fig. 4 second row) and salinity (S only; Fig. 4 third row). The former is related to the meteorological conditions, while the latter is related to the regional salt and freshwater changes. A positive PEA trend due to T or S implies a reduced vertical gradient in temperature or salinity, respectively. The decomposition reveals that in the North Sea region, both the water temperature and the salinity cause the weakening of the stratification. The changes in the trend in the Norwegian Trench results from the changing salinity trend (via freshwater inflow variability in the Baltic) (Tinker et al., 2016).
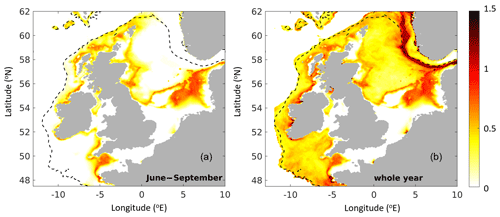
Figure 5Ratio of the number of water stratification days to the number of MHW days for (a) June to September (summer period) and the whole year. The ratio is computed with Eq. (4), using the multiyear water temperature and salinity at different depths for 1992 to 2022 (details in Table 1, ref. 1). The thin dashed line indicates the 200 m isobaths.
The sensitivity of stratification to the occurrence of marine heatwaves (MHWs), as quantified using Eq. (4), is demonstrated in Fig. 5. The illustration unequivocally indicates a profound linkage between summer stratification and MHWs, i.e., the seawater temperature T, particularly from June to September, within the southern North Sea expanse, notably in the eastern sector extending up to a longitude of 4.5° E (German Bight). This observation is consistent with the conclusions drawn by Chen et al. (2022). Another geographic area exhibiting a significant correlation between the presence of summer stratification and MHW events is the Shetland–Irish Shelf, which corresponds to region 6 in Fig. 1a. However, upon extending the temporal analysis window to encompass the entire year (i.e., all 12 months), it becomes evident that the northern North Sea (region 3; Fig. 1a), the Celtic Sea (region 8; Fig. 1a), and the Norwegian Trench (region 5; Fig. 1a) also reveal correlations with MHWs. This suggests that the manifestation of MHWs, especially those occurring during the winter season (see, e.g., Fig. 1b), instigate temperature disparities between the sea surface and its deeper layers, consequently giving rise to thermal stratification. Consequently, the escalating trend in MHW occurrences exerts an influence not only on the density stratification within the southern North Sea but also across other locales within the NWES, primarily due to the mounting incidence of MHWs during winter periods. However, in the English Channel (region 4; Fig. 1a) and the western portion of the southern North Sea (region 31; Fig. 1a) lying westward to the 4.5° E longitude, the water experiences annual thorough mixing attributed to tidal forces, thereby mitigating the impact of MHWs on density stratification.
MHW events have become more frequent and prolonged over the period 1993–2022. The total number of days experiencing MHWs showed an upward trend, particularly in the English Channel with the rate of 2–4 d yr−1. These findings align with previous studies, which reported increasing MHW occurrences globally (e.g., Oliver et al., 2018; Smale et al., 2019) and in coastal regions (Marin et al., 2021). During the past 30 years, the most prolonged MHW found in the nearshore areas is mainly attributed to the long-term changes in mean SST, which increased fastest at the coastal oceans (Marin et al., 2021). Moreover, we observed an increase in MHW duration, indicating the potential for prolonged impacts on marine ecosystems (Frölicher and Laufkötter, 2018; Suryan et al., 2021). However, the results also show that the increase in MHW frequency and length may unnecessarily coincide with the heightening of SST. In the middle North Sea and near the Danish coast, the trend of mean MHW intensity is −2 to −6 J m−3 yr−1, which appears contradictory to the increase in the MHW frequency and duration.
One possible explanation for the decrease in mean MHW intensity in the North Sea is the influence of large-scale climate patterns, such as atmospheric circulation changes. Woollings et al. (2018) demonstrated a weakening of the North Atlantic Jet Stream and an increase in atmospheric blocking events over the North Atlantic region. These changes can lead to stagnant atmospheric conditions and the trapping of warm air masses over the NWES, resulting in prolonged periods of high SST and MHW events. The increase in MHW frequency and duration may be a consequence of these altered atmospheric circulation patterns rather than a direct result of SST heightening. Furthermore, internal variability in the regional ocean, especially local processes that affect SST, play an important role (Marin et al., 2021). This includes variations in the fresh water–salt exchange and stratification (Mathis et al., 2015). Changes in these processes, particularly the Baltic Sea inflow, can affect the stability of the water column and result in a localized decrease in mean MHW intensity, while outside the North Sea region, the intensity of MHWs increases due to different oceanic processes and heat transport mechanisms in the North Atlantic Ocean (Plecha et al., 2021). In addition, it is important to note that the observed decrease in mean MHW intensity in certain areas does not negate the overall increase in MHW frequency and duration. Climate change, with its warming trends and interactions with atmospheric and oceanic conditions, is a key driver of the intensification and increased occurrence of extreme events like MHWs. While localized decreases in mean intensity may be present, the number and duration of MHW events are surpassing these reductions. These findings highlight the complex interactions between climate change, atmospheric circulation patterns, and regional oceanic processes in shaping MHW characteristics.
Despite the more frequent and prolonged MHW leading to a more stable water column, the potential energy anomaly (∅), a measure of stratification, showed a decreasing trend in the North Sea. This suggests that the MHW events and density stratification in the NWES region are not directly related. One notable piece of evidence is that the region where mean MHW intensity shows a downward trend overlaps with the area of reducing density stratification.
In general, MHW events lead to a significant increase in SST, resulting in intensified thermal stratification during these events (Chen et al., 2022). The analysis of annual mean SST trends in the NWES over the past 3 decades reveals a positive trend with a rate of change of 0.03–0.05 °C yr−1 (EU Copernicus Marine Service Product, 2023). Interestingly, the PEA due to seawater temperatures shows an opposite trend, particularly from 2003 to 2022 (Fig. 4). As this parameter quantifies the temperature heterogeneity of the water column, the decrease in PEA suggests a reduction in the temperature difference between the surface and the subsurface. With the observed increasing trend of NWES SST in response to a warming climate, the decline in PEA due to temperature can be solely attributed to the warming of the subsurface water. This warming is primarily driven by strong winter warming, leading to a weakening of the thermal stratification (Mathis and Pohlmann, 2014). This phenomenon is evident in the northern North Sea and the Celtic Sea, where the emergence of thermal stratification exhibits strong correlations with the occurrence of MHWs during winter seasons (Fig. 5). Additionally, the lower water column retains the memory of winter warming for a longer duration compared to the surface (Chen et al., 2022). Therefore, the rise in SST caused by increased MHW events is insufficient to counterbalance the overall weakening of thermal stratification due to seawater warming. Additionally, other factors and processes, such as oceanic circulation patterns and mixing mechanisms, may contribute to the observed changes in stratification (Guihou et al., 2017). Increased river runoff can lead to stronger salinity decreases at the sea surface compared to deeper layers, intensifying the stratification in terms of an increasing vertical salinity gradient (Lehmann et al., 2022). This can be seen at the estuarine zones, exemplified by the German Bight in the southern North Sea (Chegini et al., 2020). The analysis of PEA trends due to salinity also highlights the significant impact of variation in the Baltic discharge on stratification in the Norwegian Trench of the North Sea (Tinker et al., 2016).
It is notable that, within the NWES, the variability in salinity exerts a more pronounced influence on stratification compared to temperature variability. However, it is essential to recognize that climatic factors also potentially impact salinity fluctuations in the NWES. Schrum et al. (2016) reported a freshening trend in the North Sea attributed to increased river runoff and Baltic discharge, both intricately linked to an intensified water cycle and amplified net precipitation in mid- to high-latitude regions (Collins et al., 2013; Levang and Schmitt, 2015). Moreover, atmospheric heatwave events, known as significant contributors to MHW occurrences (Hobday et al., 2016), can notably affect precipitation and evaporation dynamics (Miralles et al., 2019), thereby further influencing river runoff and Baltic discharge (Lehmann et al., 2022). As a prospective avenue of research, delving into the NWES and Baltic as an integrated system would yield intriguing insights. Exploring the interplay between European continent-wide precipitation, evaporation patterns, and the evolving stratification trend in the NWES presents an intriguing prospect. Gaining comprehension of these intricate interactions would offer valuable revelations about the multifaceted mechanisms steering shifts in stratification and their intricate connections to regional climate dynamics.
Leveraging the wealth of high-resolution data furnished by the Copernicus Marine Environment Monitoring Service, we have analyzed the occurrence of MHW events and their underlying characteristics spanning the last 3 decades within the NWES region.
Our analysis revealed multiple MHW events throughout the year, including during the winter season. We find that despite showing spatial variations, MHWs have tended to become more frequent and prolonged over the past 3 decades in the NWES. The temporal dynamics of MHWs reveal a noteworthy trajectory, augmenting at a rate of 0.1 to 0.15 events yr−1 on average, contributing to a rise in annual occurrence by a range of 1 to 4 d. It is evident that coastal areas are the epicenters of this phenomenon, experiencing the swiftest augmentation in MHW duration when juxtaposed against other regions within the NWES. However, the NWES did not show a trend toward stronger stratification due to MHW occurring more frequently and lasting longer. On the contrary, it becomes less stratified, especially in the middle and northern North Sea region.
A closer examination of seawater temperature trends reveals that the rise in SST caused by increased MHW events is insufficient to offset the overall weakening of thermal stratification due to seawater warming. This is evident in the northern North Sea, where the emergence of thermal stratification exhibits a strong correlation with the occurrence of MHWs during winter seasons. However, the intricate dynamics extend beyond temperature alone. The variation in the salinity has a significant impact on the trend of change in density stratification. In particular, the influence of Baltic discharge, a veritable fulcrum of internal variability, emerges as a paramount process dictating the trajectory of changes in density stratification within the North Sea. The intricate interplay of freshwater influx from the Baltic Sea, influenced by climatic factors such as intensified water cycles and augmented net precipitation, intricately shapes the spatial distribution of salinity patterns within the North Sea realm. It is imperative to treat the NWES and Baltic as integral components of a larger, interconnected system. The interdependency between these domains necessitates a comprehensive approach that transcends arbitrary boundaries and delves into the subtle threads linking various climatic, oceanographic, and hydrological factors.
In summary, the interplay between temperature, salinity, and their intricate interactions with MHWs, Baltic discharge, and broader climatic phenomena collectively weave an intricate tapestry of density stratification trends within the North Sea region. This multifaceted narrative underscores the necessity of adopting a unified perspective that considers the complex interdependencies and feedback loops that characterize the intricate dance of nature's forces in this vital marine expanse. The outcomes of this research transcend theoretical confines, bearing practical significance for diverse sectors.
By unraveling the intricate interplay between MHWs, thermal stratification, and salinity dynamics, our study contributes to a more comprehensive understanding of climate change impacts on regional oceanic systems. The implications extend to domains such as ecosystem dynamics, fisheries, and related sectors, which are poised to be influenced by the enduring alterations in the thermal stratification patterns. These changes have far-reaching implications for the ecological and socioeconomic fabric of the NWES region.
The datasets presented in this study can be found in the CMEMS online repository. Details are listed in Table 1 of this paper.
WC conceptualized the study, analyzed data, and wrote this article. JS contributed to the writing of the article and quality control.
The contact author has declared that neither of the authors has any competing interests.
Views and opinions expressed are however those of the author(s) only and do not necessarily reflect those of the European Union or the European Health and Digital Executive Agency (HaDEA). Neither the European Union nor HaDEA can be held responsible for them.
Publisher’s note: Copernicus Publications remains neutral with regard to jurisdictional claims made in the text, published maps, institutional affiliations, or any other geographical representation in this paper. While Copernicus Publications makes every effort to include appropriate place names, the final responsibility lies with the authors.
Wei Chen acknowledges the Copernicus Marine Service Evolution Strategy 2022 project Coastal-risks: Predicting risks of the German Bight coast under extreme storm events (21036-COP-INNO SCI) and the EU Green Deal project REST-COAST: Large scale restoration of coastal ecosystems through rivers to sea connectivity (grant no. 101037097). Joanna Staneva acknowledges OLAMUR project: Offshore Low-trophic Aquaculture in Multi-Use Scenario Realisation (grant agreement 101094065). We also thank Lorena Moreira Mendez and Karina von Schuckmann for their comments and great help in improving the quality of this paper.
This research has been funded by the Copernicus Marine Service Evolution (21036-COP-INNO SCI) and the European Union (grant agreement nos. 101037097 and 101094065).
This paper was edited by Marilaure Grégoire and reviewed by two anonymous referees.
Borges, A., Royer, C., Martin, J. L., Champenois, W., and Gypens, N.: Response of marine methane dissolved concentrations and emissions in the Southern North Sea to the European 2018 heatwave, Cont. Shelf Res., 190, 104004, https://doi.org/10.1016/j.csr.2019.104004, 2019.
Chegini, F., Holtermann, P., Kerimoglu, O., Becker, M., Kreus, M., Klingbeil, K., Gräwe, U., Winter, C. and Burchard, H.: Processes of stratification and destratification during an extreme river discharge event in the German Bight ROFI, J. Geophys. Res.-Oceans, 125, e2019JC015987, https://doi.org/10.1029/2019JC015987, 2020.
Chen, W., Staneva, J., Grayek, S., Schulz-Stellenfleth, J., and Greinert, J.: The role of heat wave events in the occurrence and persistence of thermal stratification in the southern North Sea, Nat. Hazards Earth Syst. Sci., 22, 1683–1698, https://doi.org/10.5194/nhess-22-1683-2022, 2022.
Collins, M., Knutti, R., Arblaster, J., Dufresne, J.-L., Fichefet, T., Friedlingstein, P., Gao, X., Gutowski, W. J., Johns, T., Krinner, G., Shongwe, M., Tebaldi, C., Weaver, A. J., and Wehner, M.: Long-term climate change: projections, commitments and irreversibility, in: Climate change 2013: the physical science basis, Contribution of Working Group I to the Fifth Assessment Report of the Intergovernmental Panel on Climate Change, edited by: Stocker, T. F., Qin, D., Plattner, G.-K., Tignor, M., Allen, S. K., Boschung, J., Nauels, A., Xia, Y., Bex, V., and Midgley, P. M., Cambridge University Press, https://www.ipcc.ch/site/assets/uploads/2018/02/WG1AR5_Chapter12_FINAL.pdf (last access: 21 March 2024), 2013.
EU Copernicus Marine Service Product: Atlantic-European North West Shelf-Ocean Physics Reanalysis, Mercator Ocean International [data set], https://doi.org/10.48670/moi-00059, 2021.
EU Copernicus Marine Service Product: European North West Shelf Sea Surface Temperature trend map from Observations Reprocessing, Mercator Ocean International [data set], https://doi.org/10.48670/moi-00276, 2023.
Frölicher, T. L. and Laufkötter, C.: Emerging risks from marine heat waves, Nat. Commun., 9, 650, https://doi.org/10.1038/s41467-018-03163-6, 2018.
Frölicher, T. L., Fischer, E. M., and Gruber, N.: Marine heatwaves under global warming, Nature, 560, 360–364, https://doi.org/10.1038/s41586-018-0383-9, 2018.
Guihou, K., Polton, J., Harle, J., Wakelin, S., O'Dea, E., and Holt, J.: Kilometric scale modeling of the NorthWest European Shelf Seas: Exploringthe spatial and temporal variability ofinternal tides, J. Geophys. Res.-Oceans, 123, 688–707, https://doi.org/10.1002/2017JC012960, 2017.
Gupta, A. S., Thomsen, M., Benthuysen, J. A., Hobday, A. J., Oliver, E., Alexander, L. V., Burrows, M. T., Donat, M. G., Feng, M., Holbrook, N. J., Perkins-Kirkpatrick, S., Moore, P. J., Rodrigues, R. R., Scannell, H. A., Taschetto, A. S., Ummenhofer, C. C., Wernberg, T., and Smale, D. A.: Drivers and impacts of the most extreme marine heatwaves events, Sci. Rep., 10, 19359, https://doi.org/10.1038/s41598-020-75445-3, 2020.
Herring, S. C., Hoerling, M. P., Kossin, J. P., Peterson, T. C., and Stott, T. C.: Introduction to explaining extreme events of 2014 from a climate perspective, B. Am. Meteorol. Soc., 96, S1–S4, 2015.
Hobday, A. J., Alexander, L. V., Perkins, S. E., Smale, D. A., Straub, S. C., Oliver, E. C., Benthuysen, J. A., Burrows, M. T., Donat, M. G., Feng, M., Holbrook, N. J., Moore, P. J., Scannell, H. A., Sen Gupta, A., and Wernberg, T.: A hierarchical approach to defining marine heatwaves, Prog. Oceanogr., 141, 227–238, https://doi.org/10.1016/j.pocean.2015.12.014, 2016.
IPCC: Climate Change 2021: The Physical Science Basis. Contribution of Working Group I to the Sixth Assessment Report of the Intergovernmental Panel on Climate Change, edited by: Masson-Delmotte, V., Zhai, P., Pirani, A., Connors, S. L., Péan, C., Berger, S. , Caud, N., Chen, Y., Goldfarb, L., Gomis, M. I., Huang, M., Leitzell, K., Lonnoy, E., Matthews, J. B. R., Maycock, T. K., Waterfield, T., Yelekçi, O., Yu, R., and Zhou, B., Cambridge University Press, https://www.ipcc.ch/report/ar6/wg1/downloads/report/IPCC_AR6_WGI_SummaryVolume.pdf (last access: 21 March 2024), 2021.
Lehmann, A., Myrberg, K., Post, P., Chubarenko, I., Dailidiene, I., Hinrichsen, H.-H., Hüssy, K., Liblik, T., Meier, H. E. M., Lips, U., and Bukanova, T.: Salinity dynamics of the Baltic Sea, Earth Syst. Dynam., 13, 373–392, https://doi.org/10.5194/esd-13-373-2022, 2022.
Levang, S. J. and Schmitt, R. W.: Centennial changes of the global water cycle in CMIP5 models, J. Climate, 28, 6489–6502, https://doi.org/10.1175/jcli-d-15-0143.1, 2015.
Marin, M., Feng, M., Phillips, H. E., and Bindoff, N. L.: A global, multiproduct analysis of coastal marine heatwaves: Distribution, characteristics and long-term trends, J. Geophys. Res.-Oceans, 126, e2020JC016708, https://doi.org/10.1029/2020JC016708, 2021.
Mathis, M. and Pohlmann, T.: Projection of physical conditions in the North Sea for the 21st century, Clim. Res., 61, 1–17, https://doi.org/10.3354/cr01232, 2014.
Mathis, M., Elizalde, A., Mikolajewicz, U., and Pohlmann, T.: Variability patterns of the general circulation and sea water temperature in the North Sea, Prog. Oceanogr., 135, 91–112, 2015.
Merchant, C. J., Embury, O., Bulgin, C. E., Block, T., Corlett, G. K., Fiedler, E., Good, S. A., Mittaz, J., Rayner, N. A., Berry, D., Eastwood, S., Taylor, M., Tsushima, Y., Waterfall, A., Wilson R., and Donlon C.: Satellite-based time-series of sea-surface temperature since 1981 for climate applications, Sci. Data, 6, 1–18, 2019.
Millero, F. J. and Poisso, A.: International one-atmosphere equation of state of seawater, Deep-Sea Res. Pt. A, 28, 625–629, https://doi.org/10.1016/0198-0149(81)90122-9, 1981.
Miralles, D. G., Gentine, P., Seneviratne, S. I., and Teuling, A. J.: Land–atmospheric feedbacks during droughts and heatwaves: state of the science and current challenges, Ann. N. Y. Acad. Sci., 1436, 19–35, 2019.
Mohamed, B., Barth, A., and Alvera-Azcarate, A.: Extreme marine heatwaves and cold spells events in the Southern North Sea: classification, patterns, and trends, Front. Mar. Sci., 19, 1258117, https://doi.org/10.3389/fmars.2023.1258117, 2023.
Oliver, E. C., Donat, M. G., Burrows, M. T., Moore, P. J., Smale, D. A., Alexander, L. V., Benthuysen, J. A., Feng, M., Gupta, A. S., Hobday, A. J., Holbrook, N. J., Perkins-Kirkpatrick, S. E., Scannell, H. A., Straub, S. C., and Wernberg, T.: Longer and more frequent marine heatwaves over the past century, Nat. Commun., 9, 1–12, https://doi.org/10.1038/s41467-018-03732-9, 2018.
Oliver, E. C., Burrows, M. T., Donat, M. G., Sen Gupta, Alexander., Alexander, Lisa V., Perkins-Kirkpatrick, S. E., Benthuysen, Jessica A., Hobday, Alistair J., Holbrook, N. J., Moore, P. J., Thomsen, M. S., Wernberg, T., and Smale, D. A.: Projected marine heatwaves in the 21st century and the potential for ecological impact, Front. Mar. Sci., 6, 1–12, https://doi.org/10.3389/fmars.2019.00734, 2019.
Oliver, E. C., Benthuysen, J. A., Darmaraki, S., Donat, M. G., Hobday, A. J., Holbrook, N. J., Schlegel, R. W., and Gupta, A. S.: Marine heatwaves, Annu. Rev. Mar. Sci., 13, 313–342, https://doi.org/10.1146/annurev-marine-032720-095144, 2020.
Plecha, S. M., Soares, P. M. M., Silva-Fernandes, S. M., and Cabos, W.: On the uncertainty of future projections of Marine Heatwave events in the North Atlantic Ocean, Clim. Dynam., 56, 2027–2056, https://doi.org/10.1007/s00382-020-05529-3, 2021.
Pohlmann, T.: Calculating the development of the thermal vertical stratification in the North Sea with a three-dimensional baroclinic circulation, Cont. Shelf Res., 16, 163–194, 1996.
Renshaw, R., Wakelin, S., Golbeck, I., and O'Dea, E.: EU Copernicus Marine Service Quality Information Document for the Atlantic-European North West Shelf-Ocean Physics Reanalysis, NWSHELF_MULTIYEAR_PHY_004_009, Issue 5.2, Mercator Ocean International, https://catalogue.marine.copernicus.eu/documents/QUID/CMEMS-NWS-QUID-004-009.pdf (last access: 19 July 2023), 2021.
Schlegel R. W., Oliver E. C. J., and Chen K.: Drivers of marine heatwaves in the northwest atlantic: the role of air–sea interaction during onset and decline, Front. Mar. Sci., 8, 627970, https://doi.org/10.3389/FMARS.2021.627970, 2021.
Schrum, C., Lowe, J., Meier, H. M., Grabemann, I., Holt, J., Mathis, M., Pohlmann, T., Skogen, M. D., Sterl, A., and Wakelin, S.: Projected change – North Sea, North Sea Region Climate Change Assessment, Springer International Publishing, 175–217, ISBN 978-3-319-39745-0, 2016.
Simpson, J. H.: The shelf-sea fronts: implications of their existence and behaviour, Philos. T. Roy. Soc. A, 302, 531–546, https://doi.org/10.1098/rsta.1981.0181, 1981.
Smale, D. A., Wernberg, T., Oliver, E. C., Thomsen, M., Harvey, B. P., Straub, S. C., Burrows, M. T., Alexander, L. V., Benthuysen, J. A., Donat, M. G., Feng, M., Hobday, A. J., Holbrook, N. J., Moore, Perkins-Kirkpatrick, S. E., Scannell, H. A., Gupta, A. S., Payne, B. L., and Moore, P. J.: Marine heatwaves threaten global biodiversity and the provision of ecosystem services, Nat. Clim. Change, 9, 306–312, https://doi.org/10.1038/s41558-019-0412-1, 2019.
Suryan, R. M., Arimitsu, M. L., Coletti, H. A., Hopcroft, R. R., Lindeberg, M. R., Barbeaux, S. J., Batten, S. D., Burt, W. J., Bishop, M. A., Bodkin, J. L., Brenner, R., Campbell, R. W., Cushing, D. A., Danielson, S. L., Dorn, M. W., Drummond, B., Esler, D., Gelatt, T., Hanselman, D. H., Hatch, S. A., Haught, S., Holderied, K., Iken, K., Irons, D. B., Kettle, A. B., Kimmel, D. G., Konar, B., Kuletz, K. J., Laurel, B. J., Maniscalco, J. M., Matkin, C., McKinstry, C. A. E., Monson, D. H., Moran, J. R., Olsen, D., Palsson, W. A., Pegau, W. S., Piatt, J. F., Rogers, L. A., Rojek, N. A., Schaefer, A., Spies, I. B., Straley, J. M., Strom, S. L., Sweeney, K. L., Szymkowiak, M., Weitzman, B. P., Yasumiishi, E. M., and Zador, S. G.: Ecosystem response persists after a prolonged marine heatwave, Sci. Rep., 11, 6235, https://doi.org/10.1038/s41598-021-83818-5, 2021.
Tinker, J. and Howes, E. L.: The impacts of climate change on temperature (air and sea), relevant to the coastal and marine environment around the UK, MCCIP Science Review, https://doi.org/10.14465/2020.arc01.tem, 2020.
Tinker, J., Lowe, J., Pardaens, A., Holt, J., and Barciela, R.: Uncertainty in climate projections for the 21st century northwest European shelf seas, Prog. Oceanogr., 148, 56–73, 2016.
Tonani, M., Ascione, I., and Saulter, A.: EU Copernicus Marine Service Product User Manual for the Atlantic-European North West Shelf-Ocean Physics Reanalysis, NWSHELF_MULTIYEAR_PHY_004_009, Issue 1.3, Mercator Ocean International, https://catalogue.marine.copernicus.eu/documents/PUM/CMEMS-NWS-PUM-004-009-011.pdf (last access: 19 July 2023), 2022.
Wakelin, S., Townhill, B., Engelhard, G., Holt, J., and Renshaw, R.: Marine heatwaves and cold-spells, and their impact on fisheries in the southern North Sea, EGU General Assembly 2021, online, 19–30 April 2021, EGU21-7329, https://doi.org/10.5194/egusphere-egu21-7329, 2021.
Wernberg, T., Smale, D. A., Tuya, F., Thomsen, M. S., Langlois, T. J., De Bettignies, T., Bennett, S., and Rousseaux, C. S.: An extreme climatic event alters marine ecosystem structure in a global biodiversity hotspot, Nat. Clim. Change, 3, 78–82, https://doi.org/10.1038/NCLIMATE1627, 2013.
Wernberg, T., Bennett, S., Babcock, R. C., de Bettignies, T., Cure, K., Depczynski, M., Dufois, F., Fromont, J., Fulton, C. J., Hovey, R. K., Harvey, E. S., Holmes, T. H., Kendrick, G. A., Radford, B., Santana-Garcon, J., Saunders, B. J., Smale, D. A., Thomsen, M. S., Tuckett, C. A., Tuya, F., Vanderklift, M. A., and Wilson, S.: Climate-driven regime shift of a temperate marine ecosystem, Science, 353, 169–172, https://doi.org/10.1126/science.aad8745, 2016.
Woollings, T., Barriopedro, D., Methven, J., Son, S.-W., Martius, O., Harvey, B., Sillmann, J., Lupo, A. R., and Seneviratne, S.: Blocking and its Response to Climate Change, Curr. Clim. Change Rep., 4, 287–300, https://doi.org/10.1007/s40641-018-0108-z, 2018.
Zhao, Z. and Marin, M.: A MATLAB toolbox to detect and analyze marine heatwaves, Journal of Open Source Software, 4, 1124, https://doi.org/10.21105/joss.01124, 2019.