the Creative Commons Attribution 4.0 License.
the Creative Commons Attribution 4.0 License.
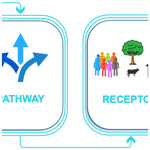
Sea Level Rise in Europe: Impacts and consequences
Roderik van de Wal
Debora Bellafiore
Paula Camus
Christian Ferrarin
Gualbert Oude Essink
Ivan D. Haigh
Piero Lionello
Arjen Luijendijk
Alexandra Toimil
Joanna Staneva
Michalis Vousdoukas
Sea level rise has major impacts in Europe which vary from place to place and in time, depending on the source of the impacts. Flooding, erosion, and saltwater intrusion lead via different pathways to cause various consequences in coastal regions across Europe. Flooding, via overflow, overtopping, and breaching, damages assets, the environment, and people. Coastal erosion leads also to damage, and saltwater intrusion affects ecosystems and surface waters and salinizes coastal aquifers, diminishing freshwater availability and causing salt damage to crops and health issues in people. This paper provides an overview of the various impacts and consequences of sea level rise in Europe.
- Article
(10280 KB) - Full-text XML
- BibTeX
- EndNote
Sea level rise (SLR) is a major threat for coastal zones, inducing hazards such as coastal flooding (mild and chronic at high tides or intense and episodic during storms), permanent submersion of coastal zones, coastal erosion, salt intrusion in surface- and groundwater (with adverse impacts on drinkable water and agriculture), problems with water management, and coastal ecosystem degradation or loss (affecting coastal wetlands and contributing to coastal zone protection, biodiversity conservation, and carbon storage). As sea level is bound to rise over the next few centuries (Melet et al., 2024), it is with great certainty that coastal zones and communities will be increasingly threatened by sea level changes at various timescales, ranging from episodic extreme events to interannual–centennial changes and trends linked to climate change and modes of variability (Hallegatte et al., 2013; Oppenheimer et al., 2019; Cooley et al., 2022; Glavovic et al., 2022; Bednar-Friedl et al., 2022). Locally, these threats can be reinforced by subsidence caused by human activities like groundwater pumping or groundwater fluid extraction for energy purposes (Carbognin and Tosi, 2002).
Impacts of SLR result from the combination of sea level changes, exposure, and vulnerability (Cardona et al., 2012). Sea level changes with a focus on Europe are discussed in Melet et al. (2024). This paper aims to describe the different impacts of sea level rise in Europe, following the physical evidence expressed by Melet et al. (2024), and is intended for local and governmental stakeholders planning on raising awareness and considering adaptation measures in their region. We attempt to provide an aggregation of the consequences in a heterogeneous landscape of information.
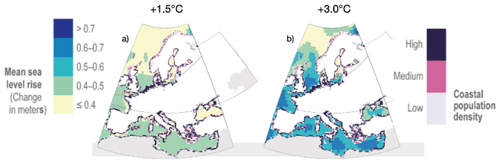
Figure 1Mean sea level rise (SLR; in m) and coastal population density in Europe for global warming levels of +1.5 and +3 °C. SLR data consider the long-term period (2081–2100) and SSP1-2.6 for (a) and SSP3-7.0 for (b). Adapted from Fig. 13.4 in Chap. 13 of AR6 WG2 (Bednar-Friedl et al., 2022).
The increased hazards posed by SLR in response to climate change have been identified as the main driver of future rise in coastal flood risks, with the relative importance of trends in exposure, related to coastward migration, urbanization, and rising asset values, diminishing over time (Vousdoukas et al., 2018a). However, population and economic activities are expected to change in Europe and worldwide as well, possibly also increasing the exposure. Coastal zones are increasingly more densely populated than the hinterland (Small and Nicholls, 2003) and exhibit higher rates of population growth and urbanization, which are concentrating economic assets and critical infrastructure. Coastal migration is driven by the combinations of specific economic, geographic, and historical conditions and includes the concentration of densely settled agricultural areas in well-watered fertile deltas and coastal plains (Hugo, 2011; McGranahan et al., 2007). It plays particularly on longer timescales but is hard to quantify. The exposure of people and assets to SLR hazards is therefore widespread and increasing and can thereby extend to a change in the vulnerability to SLR (e.g., urbanization changes the imperviousness of flood-prone areas; Andreadis et al., 2022). Figure 1 shows that the coastal zones in Europe (EU) are highly urbanized; more than 30 million people live in the 100-year event flood coastal plain and 50 million in the contiguous and hydrologically connected zone of land along the coast and below 10 m elevation (sometimes referred to as the low-elevation coastal zone (LECZ); Neumann et al., 2015). Whether the number of people exposed changes over time depends on assumptions on future developments of fertility, mortality, and migration.
A land use planning strategy in coastal lowlands can reduce exposure of the EU population to SLR (see Bisaro et al., 2024). A good example is, for instance, by developing coastal setback zones, which are buffer spaces defined by a specific distance from the shoreline's highest water mark where new developments in potentially exposed coastal regions are restricted. This can reduce the exposure of new urban development by at least 50 % in most EU countries by 2100 (Wolff et al., 2023).
Due to the large economic value of coastal zones, economic losses due to coastal flood risks are huge (Abadie et al., 2020; Hallegatte et al., 2013). Presently, the expected annual damage from coastal flooding for Europe alone is around EUR 1.25 billion but could increase by 2–3 orders of magnitude if coastal adaptation is only maintained to its current level (Vousdoukas et al., 2018a). The impacts of floods (both marine and riverine/pluvial) on people, the built environment, and the economy is one of the four key climate-change-induced risks identified for Europe (Bednar-Friedl et al., 2022). Deltas are particularly vulnerable to SLR because of, among other factors, the large population pressure. In Europe, the main deltas are those of the Rhine–Meuse–Scheldt (NL), Rhône (FR), Po (IT), and Ebro (SP) rivers. Rotterdam and London are amongst the world's most exposed cities in terms of the population living in the 100-year event flood plain if there were no flood protection (Hallegatte et al., 2013). In addition to human fatalities, economic losses due to coastal flood risks are considerable. European cities for which the annual average losses due to coastal flooding by 2050 will increase particularly, assuming present-day defense standards or flood probability, tend to be concentrated along the Mediterranean coast (Hallegatte et al., 2013). These cities (e.g., Venice) were built close to the shore since the historical sea level variability has been low (e.g., small tidal range and interannual variability); as a consequence, changes in the mean sea level are felt earlier than in regions where the sea level variability is higher.
Therefore, SLR creates risks for people, ecosystems, land uses, the built environment, and human activities (Cooley et al., 2022). In this paper, a summary of the sixth cycle of the Intergovernmental Panel on Climate Change (IPCC) report is provided (Sect. 2). Then, various impacts of SLR are discussed from a European perspective, using the source, pathway, and receptor (SPR) framework introduced in Sect. 3. SLR impacts are discussed for coastal flooding (Sect. 4), coastal erosion (Sect. 5), and saltwater intrusion (Sect. 6).
An updated assessment of the impact and risks for natural and human systems by SLR is provided by the recent Sixth Assessment Report of the Intergovernmental Panel on Climate change (IPCC AR6), whose Working Group II (WG2; Pörtner et al., 2022) assessed the impacts, adaptation, and vulnerabilities related to climate change. SLR is considered in many chapters, particularly in Chap. 3 (“Ocean and coastal ecosystems and their services”) (Cooley et al., 2022; see cross-chapter Box 3 for an overall summary) and Chap. 6 (“Cities, settlements and key infrastructure”) (Dodman et al., 2022), with more focused material presented in the cross-chapter in Paper 2 (“Cities and settlements by the sea”) (Glavovic et al., 2022). Material directly addressing European regional issues is included in Chap. 13 (“Europe”) (Bednar-Friedl et al., 2022) and in the cross-chapter Paper 4 (“Mediterranean region”) (Ali et al., 2022). The material on SLR covered by AR6 WG2 (Dodman et al., 2022) and the AR6 Synthesis Report (Core Writing Team et al., 2023) builds on and updates the work presented by Oppenheimer et al. (2019) and the AR6 reports.
There is extensive evidence that at global scale, relative SLR is already impacting ecosystems, human livelihoods, infrastructure, food security, and the climate mitigation potential at the coast (Pörtner et al., 2022). Observed impacts include chronic flooding at high tides, more frequent episodic flooding during storms, wetland and underground water salinization and ecosystem transitions, increased erosion, and coastal flood damages. SLR poses risks for cities, settlements, and populations in low-elevation coastal zones, cultural heritage along coasts, and threatens the very existence of some island nations. The large exposure caused by the disproportional concentration of population and economic activities in coastal areas leads to high risks which are very likely to increase further with future SLR. In addition, risks in the agriculture sector and nature conservation are induced by the salinization of groundwater, estuaries, wetlands, and soils. The IPCC AR6 WG2 shows that these risks will generally increase with SLR. Along many European coastlines, extreme water levels, coastal floods, and sandy coastline recession are projected to increase during the 21st century, mainly because of the increase in relative SLR.
According to the IPCC AR6 WG2, it is with great certainty that an acceleration in the SLR will increase risks to people and infrastructure from the inundation and extreme floods along European low-lying coasts and estuaries. Related damages will increase at least 10-fold even before the end of the 21st century, assuming the present levels of adaptation and mitigation measures. Annual expected damage (which today is EUR 1.3 billion) is foreseen to increase disproportionately with global warming. It is estimated to be in the range of EUR 13–39 billion by 2050 at global warming levels between 2 and 2.5 °C and EUR 93–960 billion by 2100 between a 2.5 and 4.4 °C temperature increase. Assuming the present distribution of population and protection levels, the increase in the number of people at risk depends on the emission scenario; with respect to the present, an extra 10 million people will be at risk of experiencing a 100-year flood event under a very high-emission scenario (RCP8.5) by 2100, whereas just below 10 million people will be at risk under a low-emission scenario (RCP2.6) by 2150. Along low-lying coasts and estuaries, flood risks might further increase because of compounding storm surges, waves, rainfall, and river runoff events, but to date, this has been poorly quantified (see also Sect. 4). Port operations may be negatively affected by SLR in northern and western Europe. In Mediterranean ports, the negative effects are to be expected by a combined change in wave regimes and sea level.
In the IPCC AR6 WG2, it is shown that soft cliffs and beaches in Europe are most affected by coastal erosion. Observations suggest that 27 %–40 % of Europe's sandy coast are already eroding today, although there is no convincing evidence that past erosion of sandy shorelines can be attributed to climate change or SLR. At the same time, there is a great deal of certainty that SLR will increase the sandy shoreline retreat in the future, but the actual rates are very uncertain. On a centennial timescale, coastal erosion and flooding will become an existential threat for some coastal communities and UNESCO World Heritage sites, especially in the Mediterranean region (Reimann et al., 2018; Sabour et al., 2020), where the problem of propagation of waves in ports is also foreseen in future. Moreover, seawater intrusion in coastal aquifers and surface waters is projected to increase by the combination of overexploitation and SLR, with pronounced impacts on agricultural productivity.
The IPCC AR6 WG2 shows that warming is the main climate hazard for European coastal ecosystems. However, rapid SLR (potentially aggravated by human-induced subsidence) is also expected to have negative impacts by reducing the surface area of intertidal flats (e.g., the Wadden Sea) that cannot always be compensated by sediment accumulation. By 2100 under intermediate scenarios, coastal erosion will cause the loss of 4.2 %–5.1 % of the present values of ecosystem services and reduce their contribution to shoreline protection across Europe. The vulnerability of Europe's coastal subtidal seagrass meadows and intertidal salt marshes to SLR is particularly high in the microtidal areas of the Baltic and Mediterranean coasts, with a potential loss of 75 % of Posidonia oceanic seagrass habitats in the Mediterranean Sea. In addition, non-sea-level-related coastal squeeze is also a threat which may cause ecosystem loss.
To describe the main impacts of SLR across Europe, we use the concept of the source–pathway–receptor (SPR), proposed by Sayers et al. (2002), as an alternative approach to the traditional exposure vulnerability approach (Nicholls et al., 2007). The “source” describes the origin of the event that causes flooding. The “pathway” is the route that a hazard takes to reach the “receptors” and includes processes and characteristics of the coastline influencing or mediating the hazard. The receptor is the exposed element (e.g., people, property, and environment) that may be harmed by the event and the corresponding social, economic and environmental effects on the receptors. The concept is illustrated in Fig. 2. The SPR concept will be applied to coastal flooding (Sect. 4), erosion (Sect. 5), and saltwater intrusion (Sect. 6). The SPR concept has been used in many coastal contexts, for example, by Thorne et al. (2007), Donovan et al. (2013), Villatoro et al. (2014), and Haigh et al. (2022).
The first pronounced impact of SLR that we consider is coastal flooding. A schematic overview of the SPR concept for coastal flooding is provided in Fig. 3. Coastal floods are amongst the most impactful hazards – both in Europe and globally – with wide-ranging social, economic, and environmental consequences. Many severe flooding events have affected European coastlines throughout history (Haigh et al., 2015, 2017; Paprotny et al., 2018; Ferrarin et al., 2022). An increase in the coastal flooding frequency is one of the most certain and costly consequences of SLR (Nicholls and Cazenave, 2010). Flood defense standards in many European countries are among the highest in the world (Sect. 4.2). However, significant populations and assets are in coastal flood plains and are threatened when defense infrastructure fails or is exposed to flooding exceeding the protection standard. Furthermore, the impacts of coastal flooding are likely to increase as flood defense infrastructure is aging, as the coastal population continues to grow, and as urbanization and development in low-elevation coastal zones continue (Stevens et al., 2016; McMichael et al., 2020). In addition, further decline in the extent of natural habitats (i.e., salt marshes, mudflats, shingle beaches, and sand dunes), which act as a natural buffer against flooding, has the potential to increase coastal flood risks (Campbell and Keddy, 2022).
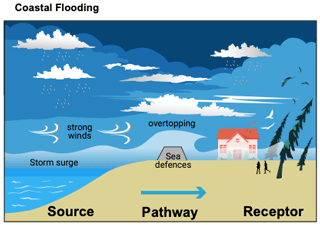
Figure 3The SPR concept visualized for flooding. Note that overtopping can also be replaced by overflow or breaching.
Multiple drivers, some related to climate change and others not, influence coastal flood risk and its future change. These different drivers can be considered using the SPR conceptual model, as explained in Sect. 3. The source of coastal floods (describing the origin of the event) is formed by extreme coastal water levels (including the contributions from tides, storm surge and wave runup superimposed on the relative mean sea level). The influence of climate change on the source component of coastal flooding is discussed in Sect. 4.1 and in Melet et al. (2024, Sects. 4.3 and 5.3). In estuaries, the compounding influence of rainfall and fluvial input can be important, leading to compound flooding, which is described as a special case in Sect. 4.2. The pathway represents how seawater makes its way onto normally dry land to cause flooding. Climate change, especially SLR, can significantly affect this pathway, in addition to modifying the source profile. This influence of SLR on pathways is discussed in Sect. 4.3. Receptors are discussed in Sect. 4.4. Initiatives to develop flood-related climate services in Europe are discussed in Sect. 4.5.
4.1 Source of flooding
Coastal floods are governed by anomalously high water levels exceeding a site-specific threshold. Extreme coastal water levels (ECWLs) arise as combinations of different drivers, namely (1) astronomical tides; (2) storm surges and associated seiches; (3) waves, including setup, infragravity waves, and swash (e.g., Dodet et al., 2019); and (4) relative mean sea level (including SLR and land subsidence) (see also Melet et al., 2024). These four components exhibit considerable intra- and interannual variability (e.g., driven by tidal cycles) (Haigh et al., 2011) or climate variability, such as the North Atlantic Oscillation (Hurrell, 1995; Mentaschi et al., 2017; Boucharel et al., 2023), or changes in wave climate (Morim et al., 2019; Melet et al., 2020) or ocean processes at basin scales (Menna et al., 2022; Meli et al., 2023). In addition, there are non-linear interactions between the four components (Horsburgh and Wilson, 2007; Idier et al., 2019; Arns et al., 2020). Long-term changes in any or all of the four components can modify the variability in the frequency and magnitude of ECWLs and thus affect coastal flooding.
ECWLs, and hence the frequency of coastal flooding events, are impacted by climate change in three main ways (Pugh and Woodworth, 2014), as also argued in Melet et al. (2024): (1) SLR affects the ECWLs directly by raising the baseline mean water level and leading to lower storm surge and/or wave elevations necessary to cause flooding; (2) rising mean sea levels alter water depths and therefore modify the propagation and dissipation of the tide and storm surge components or alter wave processes in shallow water (e.g., Arns et al., 2017; Chaigneau et al., 2023); and (3) climate variations in the tracks, speed, strength, and frequency of weather systems may alter the intensity and/or duration and frequency of storm surges and waves (and variations in rainfall and river discharge in estuaries).
As discussed in Melet et al. (2024, Sects. 4.3, 5.3), direct changes in the mean sea levels appear to have been the main driver of observed changes in ECWLs in the past (Menéndez and Woodworth, 2010; Marcos et al., 2015; Ferrarin et al., 2022) and are projected to dominate changes in extremes along the European coastline in the future, increasing the likelihood of coastal flooding (Vousdoukas et al., 2016, 2017). However, changes in storm surges (Calafat and Marcos, 2020; Calafat et al., 2022; Muis et al., 2020) and wave height (Benetazzo et al., 2022; Vousdoukas et al., 2018b; Aarnes et al., 2017) have and may also play a substantial role in the changes in ECWLs in some European regions in the future. Coastal flooding could also be influenced by changes in tides, especially in regions with a shallow water depth. Regionally coherent changes (positive and negative) in the tidal range have been observed in historic sea level records around both European and global coastlines and are projected to occur in the future with changes in water depth driven by SLR and factors such as ice sheet extent and ocean warming (Pickering et al., 2012; Ferrarin et al., 2015; Idier et al., 2017; Haigh et al., 2020). Changes in tidal range are likely to be smaller than ±15 % of mean SLR along most coastlines.
The relative contribution of SLR to changes in coastal flooding depends on different factors. Areas with small tidal (∼ 2 m) amplitude will be primarily affected by mean sea level changes. For example, in the Mediterranean Sea, extreme sea levels that are now occurring once in a century are expected to occur at a higher frequency in future, depending on climate scenarios, but in some places they are expected to occur even more frequently than annually. This intensification is stronger than the overwash and flooding in the south of Portugal, where current return periods of 1 in 100 years can reduce to lower than 1 in 20 years by 2055 and 1 in 10 years by 2100 (Ferreira et al., 2021). Venice is an exception for the Mediterranean Sea, as the existing protection measures are higher than for most of the south European coastlines and could potentially protect the monumental city during the next few decades (Lionello et al., 2021; Mel et al., 2021).
4.2 Compound flooding
Many coastal settlements along the European coastline are in estuaries and lagoons (e.g., London, Rotterdam, Hamburg, Venice, and Lisbon). In these coastal regions, floods can arise not only from climate-driven changes in oceanographic sources (e.g., tides, storm surges, and waves) but also via river discharge (fluvial) and direct surface runoff (pluvial). These mainly arise from heavy precipitation but are also incurred via snowmelt. Many cities and towns located along coastline can also experience flooding during heavy rainfall because of insufficient drainage during high tides (Van Den Hurk et al., 2015). In the past, flood risk assessments typically considered the oceanographic, fluvial, and pluvial drivers of flooding separately. However, in coastal regions, floods are often caused by more than just one factor, which can be physically correlated (e.g., with storms). Furthermore, the adverse consequences of a flood can be greatly exacerbated when the oceanographic, fluvial, and/or pluvial drivers occur concurrently or in close succession (i.e., a few hours to days apart), depending on local characteristics which influence lag times between variables. This can result in disproportionately extreme events, referred to as “compound flood events”, which do not necessarily have the same driver but coincide in time. Zscheischler et al. (2018) define compound events as “a combination of multiple drivers and/or hazards that contributes to societal or environmental risk”. Flood drivers are typically causally related through associated weather patterns (the modulator; Zscheischler et al., 2020), and therefore, it is assumed that stronger dependence between drivers increases the impact of compound floods (Wahl et al., 2015).
In recent years there has been a large increase in the number of studies that have started to investigate compound flood events in Europe. Many studies have been undertaken for specific localized regions in Europe, such as the Rhine delta, the Netherlands (Kew et al., 2013; Khanal et al., 2018); Brest, France (Mazas and Hamm, 2017); Santander, Spain (Rueda et al., 2016); Ravenna, Italy (Bevacqua et al., 2017); Venice, Italy (Ferrarin et al., 2022); and the River Trent, the Yare basin, the River Ancholme, and the rivers Taff and Ouse in East Sussex in the UK (Granger, 2001; Mantz and Wakeling, 1979; Thompson and Law, 1983; Samuels and Burt, 2002; and White, 2007, respectively). Larger-scale assessments of compound flood events have been undertaken more recently for the UK (Svensson and Jones, 2002, 2004; Hendry et al., 2019) and for Europe (Petroliagkis et al., 2016; Paprotny et al., 2018; Camus et al., 2021, 2022; Bevacqua et al., 2019, 2020b). On a quasi-global scale, which obviously includes Europe, Ward et al. (2018) and Couasnon et al. (2020) assessed the dependence between coastal and river flooding, using observational datasets and reanalysis, respectively.
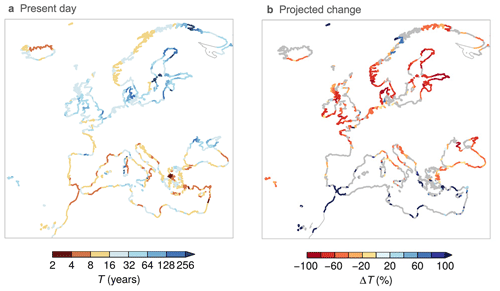
Figure 4(a) Present-day (1980–2014) probability of potential compound flooding (CF). Return periods of CF (co-occurring sea level and precipitation extremes, i.e., larger than the individual 1-year return levels) based on ERA-Interim data. (b) Future probability of potential CF multi-model mean of projected change (%) of CF return periods between future (2070–2099) and present (1970–2004) climate (Bevacqua et al., 2019).
Most of these studies quantified the statistical dependence between flooding sources as an indirect measure of the flooding hazard called compound flooding potential. The analysis of the interdependencies has been primarily limited to storm surge and precipitation (compound surge–rain events; Wahl et al., 2015; Wu et al., 2018) or to surge and river discharge (compound surge–discharge events; Ward et al., 2018; Couasnon et al., 2020; Hendry et al., 2019). In those studies, precipitation is considered a fluvial proxy, which can be assumed as an equivalent driver to discharge in small to medium-sized river catchments (Bevacqua et al., 2020a). Waves can be included as part of the total sea level by adding it linearly to the storm surge and/or astronomical tide components (Bevacqua et al., 2019). Statistical studies have notably shown that (a) the joint exceedance probability of compound surge–discharge events is on average a factor of 2–4 higher when the dependence is considered (Van Den Hurk et al., 2015; Ward et al., 2018; Santos et al., 2021); (b) ignoring the dependence between precipitation and surge can overestimate the flooding return period considerably (Bevacqua et al., 2019; Fig. 4a); and (c) the river discharge of the 50-year compound flood is up to 70 % larger, subject to the occurrence of extreme water levels (Ganguli and Merz, 2019a). Simulations of the non-linear interactions of these flood drivers at local scale using coupled modeling approaches demonstrate a rise in the extreme sea levels at some locations along the estuaries (e.g., in the Netherlands (Van Den Hurk et al., 2015) and in northwestern Spain (Bermúdez et al., 2021)). Recently, a global analysis of simulated river flood levels using a global coupled river–coast flood model framework showed that surge exacerbates 1-in-10-year flood levels at 64 % of the river mouths analyzed, with a mean increase of 11 cm (Eilander et al., 2020). Furthermore, 55 % of the world coastlines face compound storm surge and wave extremes which increase the potential coastal flooding (Marcos et al., 2019).
Although different sampling methods to identify compound events and dependence measures to quantify compound flooding potential have been used, several hotspots have been identified along the European coastlines. High joint occurrences of extreme river discharge and storm surge events are found on the coasts of Portugal, the Strait of Gibraltar, the west-facing coasts of north and central Europe (Heinrich et al., 2023b), and along the southwest coast of the UK (Hendry et al., 2019). Also, the northern and eastern Mediterranean coasts and the coast of Tunisia appear as compound flooding hotspots (Couasnon et al., 2020; Camus et al., 2021; Eilander et al., 2020). Regarding precipitation and surge, higher dependency is concentrated along the Atlantic coast and in the Mediterranean Sea (particularly in the regions of the Gulf of Valencia in Spain, northwest Algeria, the Gulf of Lion in France, the Adriatic coast of the Balkan Peninsula, the Aegean coast, southern Türkiye, and the Mediterranean–Levantine region) (Bevacqua et al., 2019; Camus et al., 2021; Fig. 4a).
Historical trends in compound flooding resulting from high coastal water levels and peak river discharge have been assessed over northwestern Europe (near the North and the Baltic seas) over 1901–2014 using 37 stream gauges (Ganguli and Merz, 2019b). Increasing trends were identified in the region from 47 to 60° N, while decreasing trends were identified along higher-latitude coasts (> 60° N).
In most regional-, continental-, or global-dependence-based analyses, compound events are identified using a two-sided conditional sampling to bivariate drivers (Wahl et al., 2015; Ward et al., 2018; Couasnon et al., 2020; Camus et al., 2021), which implies that events are either conditioned to one driver or the other. Another approach is to select pairs of high values when both variables exceed individual high percentiles (e.g., 95th percentile; as in Bevacqua et al., 2019). However, extreme water levels might be driven by events not being extreme themselves. Impact-focused approaches, modeling the relationship between extreme water levels and underlying drivers, allow the selection of large-impact events whose drivers are not necessarily extreme (Bevacqua et al., 2017; Bermúdez et al., 2019; Santos et al., 2021). Most of these studies rely on modeled data products which generally capture compound flooding but contain biases, false positive, or missed extremes (Paprotny et al., 2020).
Climate change can affect flooding dynamics through SLR, changes in each of the flood drivers, and in the interaction processes between them. However, regarding compound flooding, climate change also impacts precipitation (as average temperatures increase, more evaporation occurs, which, in turn, increases overall precipitation) and therefore river flow. SLR has been shown to increase the future compound flood hazard (Moftakhari et al., 2017; Ganguli et al., 2020; Heinrich et al., 2023a). However, uncertainties due to internal climate variability and climate model differences dominate the large uncertainty in the concurrence of flood extremes in addition to changes in the drivers (e.g., storm surges or river discharge) themselves (Bevacqua et al., 2021). Large-scale sea level/rainfall-driven compound flooding potential is projected to increase globally by more than 25 % by 2100 under the RCP8.5 scenario compared to present times (Bevacqua et al., 2020b). The probability of compound flooding due to the co-occurrence of high sea level and precipitation is projected to robustly increase (40 %–80 %) by the end of the 21st century, particularly in northern Europe, e.g., along the west coast of Great Britain, northern France, the east and south coast of the North Sea, but also along the coastlines of the eastern half of the Black Sea (Bevacqua et al., 2019; Fig. 4b). These results contrast with a predominant decrease in the compound flood hazard projected for northwestern Europe in the middle of the 21st century (Ganguli et al., 2020), which may be caused by the fact that the two studies considered different flooding drivers and multi-model ensembles.
In terms of pathways for the impact of compound flooding, elevated sea levels can block or slow down river drainage into the sea, leading to increased upstream water levels which can overflow river channels onto adjacent floodplains, thereby increasing inundation extent. Besides, floodwater can also erode riverbanks and cause breaking of the embankments, leading to further flooding. Hydrodynamic modeling is required to provide a detailed spatial mapping of water levels in estuaries or coastal river deltas. However, high-resolution hydrodynamical modeling, which includes the non-linear interaction between hydraulic processes, topography, and human interventions, is only feasible at a local scale.
In terms of receptors, historical information on past damaging floods reveals that compound events have occurred in many locations in Europe. According to the HANZE database (Paprotny et al., 2018), out of 1564 floods that occurred in 37 European countries between 1870 and 2016, 23 (i.e., 1.5 %) were identified to be compound floods recorded in six different countries (the Adriatic Sea, Italian regions of Veneto and Friuli–Venezia Giulia, the Mediterranean and the western coast of France, Ireland, UK, Belgium, and Poland). Specific examples of compound events include flooding in December 2000 in Brittany, France – 600 people were affected by a coastal flood that occurred due to the combination of heavy precipitation over several river catchments and a storm surge generated by an extra-tropical storm (Paprotny et al., 2018) – and a flood in December 1999 in Lymington, UK – the town was flooded due to tidal locking of the high runoff down the Lymington River by a large surge produced by the same storm system, with this flooding overwhelming recently upgraded defenses (Ruocco et al., 2011; Hendry et al., 2019).
4.3 Pathway for coastal flooding
Seawater tends to inundate normally dry land via three main pathways: (1) by still water simply overflowing where the water level exceeds the elevation of a natural (e.g., barrier beaches) or artificial (e.g., sea wall) barrier; (2) by waves overtopping a natural or artificial barrier; and (3) by breaching and lowering of a natural or artificial barrier, often as a consequence of prolonged overwashing or erosion at the frontal face of the barrier or by groundwater seepage (Fig. 3). Climate change, and other factors, can influence these pathways, altering local flood risk profiles. For example, SLR in regions with hard structures like barriers or dikes typically lead to a decline in the extent of natural habitats, such as salt marshes, mudflats, and sand dunes, which can act as a natural buffer to flooding (Hall et al., 2019). Decline in these natural features (and the deterioration of the flood protection infrastructure) can impact flood pathways and can increase flood hazard. In contrast, building new or maintaining and improving existing flood defenses or the application of artificial nourishment and stabilization of beaches can alter flood pathways and reduce flood risk along coasts and stabilize beaches and dunes (Haigh et al., 2022) or provide more space for water through managed re-alignment. With higher SLR, coastal flooding will progressively change due to changes in the pathway from overtopping to overflow, high-tide flooding, and ultimately permanent flooding (Ali et al., 2022).
It is increasingly recognized that natural systems that provide important buffering against floods are in decline across parts of Europe. For example, Campbell and Keddy (2022) identified a 136 (confidence interval 39–236) km2 loss of the salt marsh extent from 2000 to 2019 across Europe. Other green adaptation options include the restoration of seagrass meadows (which reduce wave height and sediment erosion) and the creation of buffer zones (Wolff et al., 2023). With mean SLR accelerating over the coming century, and thereby increasing pressure on the narrow coastal zone, there is likely to be a continued decline in the extent of natural systems (often called coastal squeeze) that contributes to natural buffers reducing coastal flood risk, which will lead to defense capital and maintenance costs increasing dramatically (Haigh et al., 2022). This is corroborated by projections of shoreline retreat along most of the global shorelines induced by SLR (Vousdoukas et al., 2020; Sect. 5) and a consequent reduction in ecosystem services (Paprotny et al., 2021). There is also concern that raising existing coastal defenses, or building new ones, will come at the cost of further biodiversity losses (Bednar-Friedl et al., 2022).
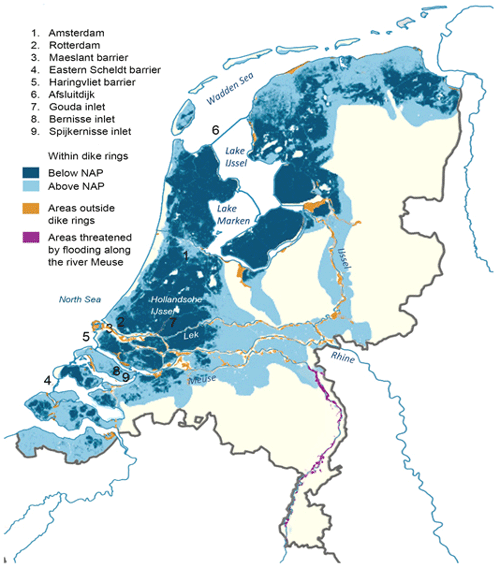
Figure 5Map of the Netherlands showing flood-prone zones (blue shading) and features of the water management system. NAP is the Amsterdam Ordnance Datum which is the reference plane for sea level height in the Netherlands. Extracted from Haasnoot et al. (2020).
Obviously, current flood risk around the coastline of Europe would be considerably higher without the decades of investment into extensive flood risk management infrastructure. Data on flood defenses over time are not well documented, but massive investments in defenses have occurred over the 20th century and early 21st century in Europe. For example, extensive flood defense infrastructure has been built in the Netherlands as part of the Delta Works, and standards of protection along stretches of the Dutch coastline now reach 1-in-10 000-year levels (Eijgenraam et al., 2014). Governing policy directives incorporate future SLR into the periodic risk assessments and defense strategy updates (Kothuis and Kok, 2017). Nearly a quarter of England's coast is now defended (Sayers et al., 2015). Around 20 movable storm surge barriers have been built around the coast of Europe since 1958, offering flood protection to millions of people and trillions of Euros of infrastructure (Mooyaart and Jonkman, 2017). This includes 6 surge barriers in Netherlands (e.g., Eastern Scheldt and Maeslant barriers; Fig. 5), 13 in the UK (e.g., Thames and Hull barriers), and 1 in Italy (the Mose barrier system for protecting the historical city of Venice and the lagoon settlements operating since October 2020), while a new surge barrier is being constructed in Belgium (Nieuwpoort). However, storm surge barriers can be used to mitigate the flood impact only in semi-enclosed coastal environments (such as bays, estuaries, and lagoons) but not along coasts facing the open sea. Extensive beach nourishment has also taken place in many European countries to counteract coastal erosion and flooding. For example, the Dutch coast is one of the most heavily nourished coasts globally (Brand et al., 2022); since 1990, more than 300 nourishment programs have taken place (including the notable Sand Engine project; Roest et al., 2021), adding an average of 12 million m3 annually to the 432 km of Dutch coastline.
However, as sea levels continue to rise, it will become increasingly costly to maintain existing flood defenses and surge barriers and to carry out coastal nourishments. In the UK, Sayers et al. (2015) showed that length of coastal defenses “highly vulnerable” to failure would almost double (triple) under 0.5 m (2.5 m) mean SLR, with the number of properties affected rising by around 160 % (490 %). Furthermore, many of the existing storm surge barriers will need to be strongly upgraded or replaced over the coming century as the sea level rises. For example, plans are underway to replace the Thames Barrier in around 2070, with options including a new barrier built farther downstream of the current one (Environment Agency, 2021). The Eastern Scheldt Barrier (Fig. 5) was designed for only 40 cm SLR, implying that a revision is needed to avoid it to be closed too often with higher sea level (Haasnoot et al., 2020), with the consequence being that the availability of maintenance windows decreases too much.
4.4 Receptors of flooding
Regarding coastal flooding, the receptor is the entity (e.g., people, property, and environment) that may be harmed by the flooding and the corresponding social, economic, and environmental consequences. The consequences associated with coastal flood events can be broadly grouped into social (e.g., loss of life, number of people evacuated, damage to residential property, or loss of cultural heritage), economic (e.g., overall monetary cost; disruptions to ports, transport, energy, public services, and water systems; and agricultural production losses) and environmental (e.g., coastal erosion and degradation or losses of coastal habitats) impacts (Haigh et al., 2017). Importantly these consequences can be long-lasting (e.g., injury or long-term physical and mental health effects; Jackson and Devadason, 2019) and can also extend outside of the coastline area directly impacted by flooding (e.g., disruption to transport or supply chains; Dawson et al., 2016). As SLR and increases in storminess enhance flood risk and its consequences, the number of receptors in flood-prone areas will grow accordingly. Changes in land use and increasing asset values in floodplains can also increase the consequences of coastal flooding (Haigh et al., 2022). In contrast, improvements in flood forecasting, early warning, emergency response, and planning can greatly reduce the consequences of flooding (Sect. 4.5). Careful spatial planning and building codes can be effective at reducing risk. Evidence from Haigh et al. (2017) suggests that the number and consequences of coastal floods have declined since 1915 in the UK, reflecting better defenses and improvements in flood forecasting, warning, emergency response, and planning (Haigh et al., 2022). As a concrete example, more than 2000 lives were lost around the coastlines of the North Sea during the flood of 1953; however, similar conditions occurring in December 2013 hardly had any societal impact, which can be attributed to these improvements and infrastructure investments (Wadey et al., 2015).
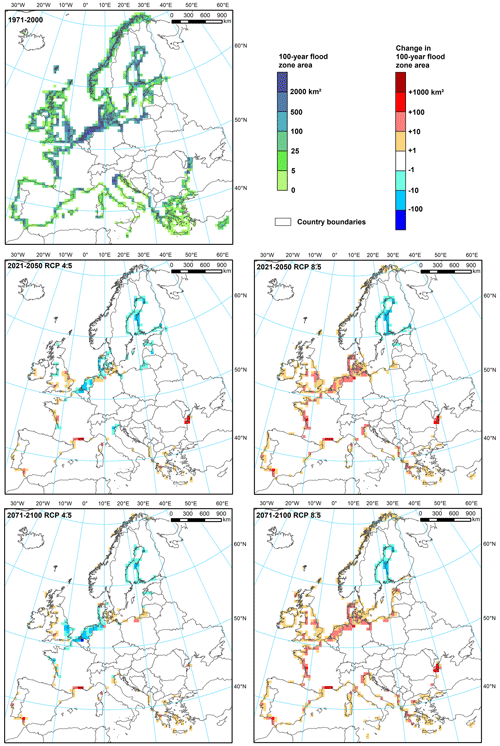
Figure 6Indication of the current area vulnerable for flooding with a return period of once in 100 years and for two different time slices under two different climate scenarios. Data are from Paprotny et al. (2018).
Paprotny et al. (2019) estimated present and future flood extents under different assumptions for coastal protection, which is a known source of uncertainty (Hinkel et al., 2021). Without considering any protection, the flooded area from the 100-year event is showing a discernible increase only towards the end of the century (6 %–10 % in average for Europe, depending on the emissions scenario; Fig. 6), and the effects of SLR are mainly demonstrated as higher flood depths. But after including coastal protection in the simulations, the flooded area is projected to increase by 10 %–15 % in 2050, depending on the emissions scenario, and by 12 %–20 % by the end of the century; for the size of the area of flooding in kilometers squared, see Table 4 in Paprotny et al. (2019) for more details. For more rare events, like a 1000-year event, SLR will drive a 48 %–67 % increase in the flood extent by the year 2100 (Paprotny et al., 2019). The country level flood extents depend on different factors, such as the exposure to extreme weather conditions, the protection standards in place, as well as the country's size and the percentage of low-lying coastal areas. For the baseline, the UK and Norway have the largest flood extent area, exceeding 4500 km2 for the 100-year event for each country. This is a result of their long coastlines exposed to intense weather conditions. Denmark and Germany follow with values around 3000 km2, and for these cases, the main driver is the flat and low-lying configuration of the coastal zones. Other countries with flood extents slightly below 2000 km2 are Greece and Italy, both characterized by long coastlines.
It is noteworthy that also low-flood-level events (i.e., nuisance flooding; Moftakhari et al., 2018) may have high impacts from the disruption of everyday routine activities and property damages, especially in lowland settlements. SLR is expected to increase not only the frequency of ECWLs but also the frequency of low-level floods, when the astronomic tide will become a major driver of flood events (Ferrarin et al., 2022). Towards the end of the century, SLR will also result in permanent flooding of certain areas. For example, in the Balearic Islands, and by the year 2100, 7.8–27.7 km2 and up to 10.9–36.5 km2 will be permanently lost to SLR under RCP4.5 and RCP8.5 emission scenarios, respectively (Luque et al., 2021).
Pan-European assessments of future coastal flood risk show general trends and allow for regional comparisons but also come with large uncertainty due to data scarcity and non-stationary conditions (Hinkel et al., 2021; Vousdoukas et al., 2018b) and cannot replace local studies (Paprotny et al., 2019). For example, flood assessment results are very sensitive to the coastal protection standards assumed which are largely unknown along most of the European coastline (Scussolini et al., 2016). Another crucial factor is the digital elevation data. Since several countries lack high-resolution lidar data, many assessments are based on less accurate global datasets, which come with vertical biases exceeding the extent of anticipated SLR (Bove et al., 2020; Kulp and Strauss, 2018; Yamazaki et al., 2017). Despite these limitations, all known flood risk assessments highlight an increase in the flooded area during the century, which also accelerates as SLR gathers pace.
4.5 Initiatives to develop flood-related climate services in Europe
The EU Adaptation Strategy emphasizes the significance of climate services in adapting to climate change. As defined by the Global Framework for Climate Services (Hewitt et al., 2012) of the World Meteorological Organization (WMO), “Climate services provide climate information to help individuals and organizations make climate smart decisions”. According to the European Commission's Roadmap for Climate Services (European Commission, 2015) definition, climate services cover
the transformation of climate-related data – together with other relevant information – into customized products such as projections, forecasts, information, trends, economic analysis, assessments (including technology assessment), counseling on best practices development and evaluation of solutions and any other services in relation to climate that may be used for the society at large. (Galluccio et al., 2024)
Information on past and future sea level change close to the coastline is being made available but still suffers from various limitations (e.g., resolution, incomplete physics in models, and scarcity of observations). In parallel, core European services have been put in place. Yet, authoritative, consistent, and decision-oriented climate services to support policies and decision-making with SLR are still in their early development worldwide.
The European Union's Earth observation program (Copernicus) monitors our planet and its environment for the ultimate benefit of society. This includes the monitoring of sea level changes and the provision of ancillary fields needed to assess coastal SLR risks, as well as the transformation of the wealth of satellite, in situ, and integrated numerical model information into added-value datasets and information usable by scientists, managers, decision-makers, and the wider public to guide adaptation and to support related policies and directives (Melet et al., 2021).
New initiatives in the framework of dedicated European research projects in the Horizon Europe program are also underway, for instance, the Coastal Climate Core Services (CoCliCo) project. CoCliCo aims to deliver an open web platform that will provide up-to-date information on present and future SLR and its impacts to support decision-making on coastal flood risk management and adaptation. The platform will grant access to the latest and consistent hindcasts and projections of sea level, process-based coastal flood maps and shoreline change estimates, flood exposure, and vulnerability information, as well as adaptation strategies and options. Users of the platform will be able to visualize, download, and analyze high-quality geospatial information layers encompassing multiple decision-oriented coastal risk scenarios. For shorter timescales, early-warning systems (EWSs) are integrated systems allowing a real-time monitoring of potential natural hazards, issuing natural hazard warnings with a few days of lead time. Informing the relevant stakeholders (e.g., civil protection agencies, regional and local authorities, and environmental agencies) is part of an integrated risk management procedure to mitigate risks. EWSs can play a critical role in classical disaster risk management cycles, supporting the preparedness and response phases, including the deployment of emergency measures for rapid response after a disaster, as well as longer-term damage assessment after the occurrence of an event. EWSs are an efficient adaptation measure by providing more than a 10-fold return on investment (Global Commission on Adaptation, 2019). The H2020 European Copernicus Coastal Flood Awareness System (ECFAS) project aims at contributing to the evolution of the Copernicus Emergency Management Service (CEMS) by demonstrating the technical and operational feasibility of a European coastal flood awareness system. Such a system will complement the existing early-warning system of the CEMS for river/pluvial floods, CEMS–EFAS (European Flood Awareness System), by adding a pan-European marine coastal flood awareness system and by tackling coastal resilience to climate risk (marine storminess and exposure). ECFAS provides an integrated risk cycle monitoring and management service from water level forecasts at the coast with a 5 d lead time (Irazoqui Apecechea et al., 2023) and rapid mapping of coastal floods and impacts on population and assets (Le Gal et al., 2022) to Risk and Recovery Mapping (RRM) for adding coast-targeted products in the aftermath of a marine flood event (e.g., shoreline displacement and maps of flooding and damages). At a national scale, the Norwegian mapping authority developed a web tool for inundation mapping, showing extreme still water levels and the projected sea level, including statistics on the areas, roads, and buildings affected now and in the future (Breili et al., 2020; https://www.kartverket.no/en/at-sea/se-havniva/se-havniva-i-kart, last access: 14 July 2024). The web tool includes statistics on the areas, roads, and buildings affected now and in the future. The German Sea Level Monitor (https://meeresspiegel-monitor.de/index.php.en, last access: 14 July 2024) provides observed and projected changes at tide gauges along the German North Sea and Baltic Sea coast.
5.1 Definition and drivers of coastal erosion
Coastal erosion is the permanent loss of land to the sea. The coastal zone hosts a wide variety of systems, like estuaries, lagoons, barrier islands, sandy and gravel beaches, dunes, cliffs, rocky shores, and built areas. The morphological changes in each of the above environments can take place at different timescales and are driven by a wide range of natural and anthropogenic factors (Mentaschi et al., 2018). Sandy beaches are the most common beach typology (Davenport and Davenport, 2006), occupying 31 % of the ice-free global coastline (Luijendijk et al., 2018). They are also particularly prone to erosion (EUROSION, 2004), especially given the fact that human development has deprived coastal systems from their natural capacity to accommodate, or recover from, erosion (Small and Nicholls, 2003). In addition, human interventions and dams tend to prevent terrestrial sediments from reaching the coastline, which favors coastal erosion (Milliman, 1997). As a result of the above, Europe's beaches have been eroding (EUROSION, 2004; Masselink et al., 2022), a trend which is projected to accelerate with climate change and SLR (Vousdoukas et al., 2020).
One of the consequences of erosion is shoreline change, which is the combined result of numerous factors, such as wind and wave climates, terrestrial sediment supply, geological control, and human interventions, among others. There is a clear cause-and-effect relationship between increasing sea levels and shoreline retreat (Bruun, 1962), which justifies the eroding trend reported by large-scale (Hinkel et al., 2013; Vousdoukas et al., 2020), regional (Toimil et al., 2017; Alvarez-Cuesta et al., 2021), or local-scale studies (Alvarez-Cuesta et al., 2021; Toimil et al., 2021; de Santiago et al., 2021; Luque et al., 2021; Romagnoli et al., 2022). Negative sediment budgets can be another factor driving a robust erosion trend, especially at sandy beaches (López-Olmedilla et al., 2022). The former can be the result of several potential natural or anthropogenic factors. In addition, several of the observed changes can relate to quasi-periodical climatic patterns affecting the wave regime (e.g., Barnard et al., 2015).
Apart from major drivers like the ones mentioned above, beach erosion and accretion often depend on a delicate balance of contrasting forces with very similar amplitudes and are therefore very difficult to predict. This applies to all scales; i.e., each wave can transport sand both towards and away from the coast (Vousdoukas et al., 2014), while storms drive erosion that can be followed by complete or partial recovery (e.g., Kroon et al., 2008; Lee et al., 1999). Moreover, the impact of extreme storm events is very much controlled by the initial beach morphological state and thus by meso- to macroscale processes (e.g., Qi et al., 2010; Vousdoukas et al., 2012). In this section, the source, pathway, and receptor are discussed for coastal erosion in Sect. 5.2. Monitoring methods, including field surveys, video monitoring, and Earth observation, are discussed in Sect. 5.3. Historical shoreline changes in Europe are summarized in Sect. 5.4, while projected shoreline changes are summarized in Sect. 5.5. Finally, interactions between coastal erosion and coastal flooding are addressed in Sect. 5.6.
5.2 SPR for coastal erosion
5.2.1 Source for erosion
Coastal erosion shares the same sources as coastal flooding; e.g., all of the components that drive ECWLs (tides, storm surges, waves, and SLR) can drive coastal erosion (Fig. 7). In addition, given that coastal erosion is strongly related to the sediment budget, sediment sources and sinks are very important. As a result, human development and dams play an important role as they tend to prevent terrestrial sediments from reaching the coastline, thus favoring coastal erosion (Anthony et al., 2019; Milliman, 1997; Meli and Romagnoli, 2022). Other natural factors which can deplete sediment from the subaerial beach are the occurrence of submerged canyons or rocky scarps in the nearshore that can act as natural barriers to onshore sediment transport (Bosserelle et al., 2021; Vousdoukas et al., 2009).
5.2.2 Pathway for erosion
Sandy beach erosion takes place when the sediment budget of a given area becomes negative. Understanding coastal change is a very challenging task since erosion or accretion is a result of multiple factors like (i) processes that take place in various temporal and spatial scales (Kroon et al., 2008; Larson and Kraus, 1994); (ii) the presence of various features which emerge through complex self-organization processes often linking different scales and processes (Murray et al., 2009; Werner, 1999); (iii) the intrinsic uncertainty in predicting the intensity and frequency of extreme events, as well as the related beach morphological response (Coco et al., 2014; Vousdoukas et al., 2012); and (iv) the high complexity of long-term processes like sediment transport and vertical land motion, which are also interconnected with, among others, geological and meteorological phenomena (Gallop et al., 2011; Vousdoukas et al., 2007), as well as human interventions which are increasing in number and extent (Luijendijk et al., 2018; Mentaschi et al., 2018). In principle, coastal erosion can be the result of any process that alters the sediment transport patterns. This can be hydrodynamic (changes in wave intensity or direction, sea level, etc.) (Sierra and Casas-Prat, 2014), related to the presence of obstacles like hard structures (Loureiro et al., 2012; Noble, 1978), or factors that affect the erodibility of the coast (Feagin et al., 2019).
5.2.3 Receptor and consequences for erosion
Coastal erosion is the process by which the land is worn away and is permanently submerged in water and can take many forms, such as the loss of sand dunes, cliffs, or beaches and can have several consequences. Among them are the destruction of buildings, roads, and other infrastructure located near the coast.
Moreover, coastal erosion can have significant impacts on the environment, economy, and human health and safety as it can lead to habitat loss for coastal species, reduce the recreational value of beaches, and increase the risk of flooding and storm damage for coastal communities.
5.3 Monitoring methods for coastal erosion
Coastal monitoring is crucial to gaining a better understanding of the likely impacts of climate change at the coast. While the long-term implications of SLR have received considerable attention, Nicholls et al. (2007) point out that more attention needs to be given to finer temporal and spatial scales, including the localized impacts of potential changes in wave climate and storminess regimes. However, monitoring the coast at higher temporal (daily to decadal) and spatial (three-dimensional) resolutions presents many challenges. Conventional survey techniques for ongoing beach surveys are both costly and labor-intensive, and meaningful trends typically require several years of data to emerge (Short and Trembanis, 2004). Currently, only half a dozen multi-decadal, high-resolution coastal monitoring programs are in operation worldwide, with few examples in Europe, including Truc Vert (France) since 2005, the Emilia–Romagna coast (Italy) since 1983, and Noordwijk (the Netherlands) since 1964.
The various monitoring methods for coastal erosion are described briefly in Table 1, highlighting the evolution from traditional to present-day monitoring techniques.
5.4 Historical shoreline change
A comprehensive European study of coastal erosion collected and analyzed aerial photographs and local surveys up to 2002 to estimate coastal erosion at the scale of Europe (EUROSION, 2004). About 20 % of the European Union's coastline suffered serious erosion impacts, with the area lost or seriously impacted estimated at 15 km2 yr−1. More recent studies at the continental to global scale confirmed that large stretches of the European coast are suffering from erosion. Using freely available optical satellite images captured since 1984, in conjunction with machine learning and image analysis methods, the shoreline changes have been mapped at a global scale (Luijendijk et al., 2018; Mentaschi et al., 2018). The application of an automated shoreline detection method to the sandy shorelines resulted in a global dataset of shoreline change rates for the 33-year period between 1984–2016 (Luijendijk et al., 2018). Analysis on satellite-derived sandy beach detection reveals that about 35 % of the European coastline is sandy, which agrees largely with the 40 % EUROSION estimate (EUROSION, 2004). Analysis of the satellite-derived shoreline data indicates that 22 % of the European sandy beaches are eroding at rates exceeding 0.5 m yr−1, while 26 % are accreting and 52 % are stable. This means that in Europe a total of more than 8200 km of sandy beaches have significantly retreated over the last decades. Areas that experience severe erosion (and accretion) are found at various locations across Europe (see Fig. 8). About 4 % of the European sandy beaches experience erosion rates classified as severe (> 3 m yr−1). Erosion rates exceed 5 m yr−1 along 2 % of the sandy shoreline. The statistics of Europe are rather similar to the global statistics stating that 24 % (28 %) of the world's beaches are eroding (accreting) (Luijendijk et al., 2018). It is important to highlight that several of the accreting or stabilizing trends found in Europe are due to human interventions, either through beach hardening or nourishment projects (Lansu et al., 2024).
5.5 Future shoreline change
Mediterranean beaches are more susceptible to the negative effects of SLR because they are narrower as a consequence of the lower tidal range and milder wave climate. This is highlighted by both large-scale (Vousdoukas et al., 2020) and regional-scale projections (Monioudi et al., 2017). For example, a recent study in the Balearic Islands projects at least 20 % of the islands' beaches losing more than 50 % of their surface by the end of the century, even if greenhouse gas emissions are mitigated (Luque et al., 2021). But even projections along the Atlantic coast report shoreline retreat, e.g., in the range of 10–45 m under the middle-of-the-road (RCP4.5) scenario and 14–66 m under the very high-emission (RCP8.5) scenario by the year 2100 for 150 km of Basque coast (de Santiago et al., 2021). A recent pan-European study projects a mean SLR-driven median shoreline retreat of 97 m (54 m) under RCP8.5 (respectively, under RCP4.5) by the year 2100. This retreat translates to 2500 km2 (1400 km2) of SLR-driven coastal land loss (Athanasiou et al., 2020; Fig. 9). Given the complexity of coastal morphodynamics, projections of shoreline changes come with high uncertainties. The future variability in wave forcing is more prominent until 2060 with regard to uncertainties, whereas after that year the uncertainties in predicting sea level rise become dominant (D'Anna et al., 2021, 2022). Of similar amplitude is also the uncertainty associated with the choice of geophysical datasets in continental-scale assessments (Athanasiou et al., 2020), reaching 45 % (26 %) of the variance in coastal land loss projections for Europe by 2050 (2100). Among the major sources of uncertainty is the geological control that can be expressed through the coastal slope (Thiéblemont et al., 2019) or through the presence of hard impermeable structures that can limit shoreline retreat to values that are substantially lower than the projected ones (e.g., Lansu et al., 2024). Even if certain future erosion risk assessments take such effects into account, by considering the spatial distribution of hard surfaces near the coast (e.g., Paprotny et al., 2021; Vousdoukas et al., 2022), this information is only available for the present, and future trends of coastal squeeze are difficult to predict (Silva et al., 2020).
5.6 Coastal erosion and flooding interactions
Coastal erosion and flooding are extremely interrelated impacts that influence each other (Sallenger, 2000; Pollard et al., 2019; Leaman et al., 2021). Erosion is a physical phenomenon through which sand is removed from the shoreface and deposited elsewhere, usually offshore. Erosion and deposition processes can change the shoreface, which affects coastal flooding caused by high water levels. In turn, these high water levels can cause further erosion or deposition. This feedback manifests itself on different timescales. In the short term, coastal morphology plays a significant role in wave energy dissipation, the total water levels reaching the coast, dune breaching, and subsequent flooding. SLR is expected to increase the frequency of episodic erosion and flooding, which in turn could be altered by changes in storminess. At longer timescales, SLR is expected to drive permanent erosion and inundation (Nicholls and Cazenave, 2010; Cazenave and Le Cozannet, 2014). This effect could be compensated or enhanced in areas with intense alongshore gradients in longshore sediment transport or chronic fluvial sediment supply. Higher water levels cause wave-driven erosion to occur higher up in the profile, resulting in net erosion and deposition on the nearshore bottom (Bruun, 1962). Additionally, deeper water reduces wave refraction and allows waves to get closer to the shore before breaking (Arns et al., 2017; Chaigneau et al., 2023), leading to increased flooding. A coastline subject to sustained erosion over time may lead to the loss of natural flood defenses (Toimil et al., 2023a, b).
The need for the joint modeling of flooding and erosion has long been recognized in the literature (Bilskie et al., 2014; Passeri et al., 2015a; Lentz et al., 2016). However, most studies to date continue to analyze these two impacts separately because of the complex relationship between driving processes and morphological response (Pollard et al., 2019; Toimil et al., 2020, 2023a). Amongst the studies that couple flooding and erosion, most of them have typically focused on historical events without considering climate change (McCall et al., 2010; Gharagozlou et al., 2020; Van Ormondt et al., 2020). These studies have primarily been conducted at the storm scale and have used pre- and post-storm topo-bathymetry data to simulate erosion and breaching using a hydro-morphodynamic model. Following the same modeling approach but in the context of climate change, some studies have incorporated the effect of SLR on hydrodynamic forcing conditions (Passeri et al., 2018; Grases et al., 2020). Sanuy and Jiménez (2021) presented a more recent application in the Tordera Delta (Spain) where the baseline topo-bathymetry was modified to consider medium-term erosion.
Studies that consider long-term shoreline changes in flooding follow more diverse approaches. Stripling et al. (2017) delivered flood maps considering long-term changes in seawall toe levels along the west coast of Calabria (Italy) and in an idealized coastal stretch around Holderness (UK). As for climate change studies, Dawson et al. (2009) developed a methodology to account for shoreline changes in coastal flood projections along the East Anglian coast (UK). Dawson et al. (2009) linked coastal flooding due to storms and SLR with long-term erosion by adjusting the likelihood of flood defense structure failure based on shoreline changes. Other studies have examined the effect of shoreline changes on coastal flooding considering an empiric (Grilli et al., 2017) and surveyed profile translation due to SLR (Barnard et al., 2019) and also due to SLR and changes in the sediment budget (Passeri et al., 2015b, 2016). Also using real profiles, Toimil et al. (2023a) proposed a suite of numerical and statistical models to analyze the influence of storm morphodynamics, SLR, erosion, and longshore sediment transport on total water levels and coastal flooding along a 40 km coastal stretch in the Spanish Mediterranean.
Current studies highlight the need to consider the interconnections between hydrodynamics and morphodynamics to better understand the functioning of the coastal system. Changes in topographic representations can alter the path and pattern of maximum water levels (Bilskie et al., 2014). Toimil et al. (2023a) found that total water levels are mainly affected by storm erosion and profile geometry and that long-term erosion is the main shoreline change contributor to the flooded area.
To date, existing research in the EU focuses on the eastern Mediterranean and North Sea basins. In the eastern Mediterranean, studies encompass a wider range of coastal typologies than in the North Sea, including cliffs, deltas, and beaches with varying levels of anthropization. Most studies model the interaction of medium- and long-term coastal changes with episodic flooding, but few additionally consider storm erosion as a flood enhancer (Toimil et al., 2023a, b). This can be particularly important for extreme weather events of high return periods, regardless of the type of coastline. The largest gap lies in studies that combine the interaction of hydro-morphological processes under climate change scenarios, as such studies are very limited in both basins. However, knowledge on the potential effects of these interconnections and the enhancing role of SLR is key information for decision-makers to make informed decisions about the future management of coastal communities.
6.1 Processes and monitoring of saltwater intrusion
Saltwater intrusion (SWI) represents the increased extent of the mixing zone between inland freshwater and saltwater (source), therefore increasing the salt content both in surface waters and in groundwaters (pathway). SWI can hinder the use of water for agriculture due to salt damage to crops (e.g., Maas and Hoffman, 1977), for ecology in freshwater wetlands (Herbert et al., 2015), and can threaten coastal communities that rely on freshwater supplies for their livelihood (receptor) (e.g., drinking water that is too salty can lead to cardiovascular diseases; He and MacGregor, 2011; see Fig. 10).
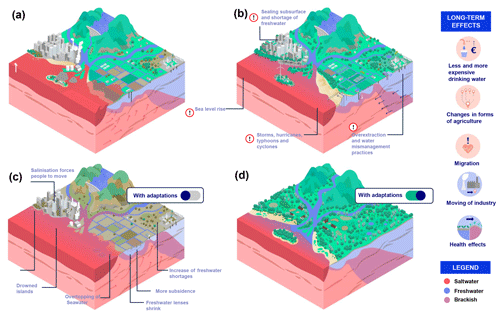
Figure 11Salinization of (ground)water resources and land for different stages of progressive development. Saltwater is represented in red/pink, brackish water in purple, and freshwater in blue. (a) Situation in the distant past, before human settlements. (b) Current situation, with effects due to climate change and human activities. (c) Situation in the future without adaptation. (d) Situation in the future with adaptation. Source: Deltares (2023).
Rivers and aquifers (good permeable water-bearing porous media) contaminated by high salinity decrease freshwater storage and water quality, reduce soil fertility (e.g., Qadir et al., 2014; Russ et al., 2020), impact on vegetation and freshwater species (Wicke et al., 2011), and affect human health. Moreover, in deltaic regions, SWI can also negatively impact ecosystem services and aquaculture activities such as clam or shrimp farming (e.g., Dierberg and Kiattisimkul, 1996; Hou et al., 2022) (Fig. 11). SWI is a slow but increasingly present hazard affecting European coasts, especially in deltas, islands, and estuaries. In these low-elevation coastal zones, climatic changes (including sea level rise) combine with the changes induced by human activities such as reducing river flows causing salt wedge shifts (Maselli and Trincardi, 2013). Figure 11b shows several processes causing problems induced by SWI such as extracting groundwater, creating controlled low-lying areas (polders), and reducing in urbanized area freshwater depletion into the groundwater systems (sealing). As a result of climate change (and associated SLR) and human-induced activities, impacts of SWI are likely to increase (e.g., Befus et al., 2020; Oude Essink et al., 2010; Zamrsky et al., 2024). In this section, we discuss the main impacts of SWI, again using the source (Sect. 6.2), pathway (Sect. 6.3), and receptor/consequence (Sect. 6.4) framework.
The scarcity of data often poses a challenge for the sustainable management of groundwater resources worldwide. Mapping and monitoring the spatial extent of near-coastal fresh groundwater resources usually requires detailed information for large coastal regions, which is often not available, whereas in situ measurements are time- and labor-consuming. To address this challenge, remotely sensed data can be a cost-effective way to gather both surface and groundwater information, covering a large area in a short period. For instance, airborne electromagnetic geophysical methods are particularly useful for detecting groundwater salinity affecting the conductivity of the groundwater. Such methods have been executed in Denmark (Duque et al., 2022), Germany (Siemon et al., 2015), and the Netherlands (Delsman et al., 2018).
6.2 Source of saltwater intrusion
Surface SWI threatens water resourcing and freshwater availability, especially in low-lying coastal areas such as deltas and estuaries (van Engelen et al., 2022), which are characterized by natural complex interactions between fresh and saline waters (Horner-Devine et al., 2015; Valle-Levinson, 2010). The inland intrusion of saline waters along the river courses is controlled by the forces acting at both the river and the sea domains, namely the advective dispersion associated with the river flow, the steady shear dispersion associated with the estuarine exchange flow, and the tidal pumping (Lerczak et al., 2006). The balance among these forces is regulated mostly by the combined action of river discharge and sea level oscillations (Bellafiore et al., 2021). Surface heat fluxes (evaporation) and the salt content in corresponding seawater also play a role in determining the extent of surface SWI. Therefore, while more evident in drought conditions (e.g., the extended 2022 drought in the Po Valley – Italy; Bonaldo et al., 2023), the processes regulating SWI and the concurring effects cannot be attributed just to one single driver. Moreover, these natural drivers can act both on short timescales, as tidal fluctuations, storm surges, and hurricanes, and at the long timescales, as climatic ,fluctuations, subsidence. and, among others, SLR. In general, the variation in surface SWI does not only affect the extension of the affected area but also can lead to the predominance of some hydrodynamic processes, for example, shifting the system from a diffusive- to an advective-dominated river dynamics (e.g., the Po river). Also, a modification of the environmental conditions in transitional areas can vary the extent of eury-, poly-, meso-, and oligohaline areas (Rodrigues et al., 2019). The concurring processes affecting surface SWI are linked to progressive river discharge decrease, increased surface heat fluxes (evaporation), and increase in the salt content and in the relative sea level. In Europe, several estuarine and deltaic systems are suffering from the progressive increase in surface SWI. Not surprisingly, this process occurs both in micro- and macro-tidal environments (e.g., the Po river and Elbe Estuary, respectively), with different sea salinity values (ranging from less haline ones in the Atlantic or North Sea to the more haline ones in the Mediterranean) and higher or lower surface heat fluxes. These environmental conditions trigger the predominance of one of the several saltwater drivers. As an example, the Tagus Estuary is exposed to a tidal excursion of up to 3.8 m, which is also amplified by resonance. Therefore, even in normal conditions, saltier seawater intrudes, affecting 43 % of the estuary area (intertidal zone). The combination of tides and the periodic exposure to droughts, with several low discharge events, seem to massively affect the system (Rodrigues et al., 2019).
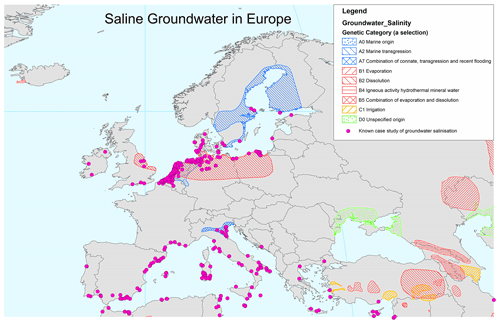
Figure 12Case studies (not exhaustive) for which the groundwater salinization is occurring in the coastal zone around Europe (updated and after Post et al., 2018, and Van Weert et al., 2009), where it may cause multi-faceted impacts combined with sea level rise changes.
The European coast already includes several cases of increased saltwater intrusion in the groundwater system (Fig. 12). Studies on the quantification of effects of changed drivers show their relative effect. In the Minho and Lima estuaries, in the northern coast of Portugal, the future SLR scenarios identify a progressive increase in saltwater extension, and the effect of the most extreme SLR scenarios is leading to a transgression of the saltier front of several kilometers (Pereira et al., 2022). In this case, SLR is identified as the dominant driver for increased saltwater intrusion when compared to future river discharge reduction. On the other hand, the quantification of the relative effect of SLR and reduced river discharge in future climate change scenarios leads to opposite conclusions in a microtidal Mediterranean system, such as the Po river delta. River discharge reduction affects SWI more than SLR (Bellafiore et al., 2021). SLR and climate-change-induced salinization are also predicted to worsen in several coastal locations in the North Sea (e.g., the Netherlands – Bonte and Zwolsman, 2010; Belgium – Bertels and Willems, 2022). In all deltaic and estuarine systems, the evaluation of changes in drivers should be carried out, considering possible additional long-term modifications of the morphologic environment, for example, due to subsidence.
Added to these natural factors and often acting in combination with them, several anthropogenic activities can exacerbate SWI by lowering the surface freshwater supply. Examples are changes in land use and land drainage, irrigation, hydropower production, and over-exploitation of coastal aquifers.
6.3 Pathway for saltwater intrusion
For SWI, the pathway reflects how seawater reaches coastal freshwater resources and causes their salinization. In estuaries and deltas, river branches are the preferential way to transport seawater upstream – through salt wedge intrusion along the riverbed and by lateral inflow of the river into the aquifers. Land salinization and aquifer contamination can also result from coastal inundation with saline waters through floods induced by storm surges (Cantelon et al., 2022). The coastal morphology and geological characteristics are therefore strongly influencing the saltwater pathway and determining complex hydrogeological interactions between groundwater, surface water, and marine water. As explained in Sect. 6.2, climate change may enhance SWI drivers by raising the mean relative sea level, as well as by reducing the net freshwater supply in rivers and aquifers. However, as for coastal flooding, SLR will also shorten the pathway of saltwater to reach land and freshwater resources by squeezing the coastline, thereby facilitating coastal inundation and intrusion of marine waters. In addition, several anthropogenic activities exacerbate SWI by altering the river mouth and coastal morphology, such as diversions of waterbodies, river channel deepening, salt marsh reduction, and human-induced subsidence (White and Kaplan, 2017) (Fig. 11).
Several prevention and adaption measures have been undertaken in the last few decades to limit coastal inundation and ingression of saline waters along the river channels and the aquifers in Europe. Anthropogenic interventions can affect SWIimpacted areas by increasing the downstream flow of freshwater (e.g., river diversion, optimization of freshwater withdrawals, and deliveries) or by preventing the upstream transport of saline water. All of the flood barriers and measures limiting land inundation described in Sect. 4 have the co-benefit of limiting the salinization of soil and intrusion of saltwater into surface and groundwater systems. The most adopted engineered strategy to prevent salt wedges from intruding in estuaries and deltas is the installation of (often submerged) mechanical barriers (gates, dams, dikes, and levees) near the river mouth that physically block the upstream flow of saline water (White and Kaplan, 2017; InCom WG, 2021). However, salt barriers are regularly damaged and breached during floods and are not effective during extreme droughts when the saline layer occupies the largest portion of the water column.
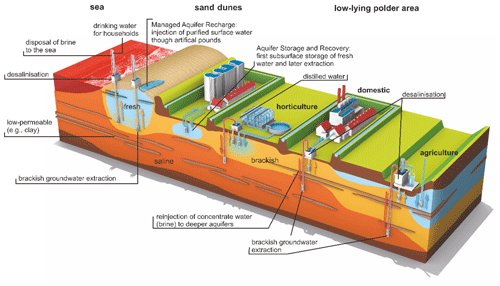
Figure 13Concept proposed for managing water resources in coastal areas, ensuring a reliable supply of freshwater for domestic, industrial, and agricultural purposes. To store excess freshwater, large-scale coastal managed aquifer recharge (MAR) and aquifer storage and recovery (ASR) methods can be used (see the text). Additionally, brackish groundwater can be intercepted to prevent the salinization of aquifers and low-lying areas, while also providing a source of freshwater after desalination (after https://www.coastar.nl/, last access: 14 July 2024).
Regarding groundwater, subsurface barrier walls (such as sheet piles, clay trenches, and injection of chemicals) are considered one of the most effective methods for inhibiting SWI (Armanuos et al., 2020). Various coastal managed aquifer recharge (MAR) schemes can be implemented to mitigate groundwater salinization in the coastal zone (Dillon et al., 2019; Oude Essink, 2001) (see e.g., Fig. 13). Sprenger et al. (2017) identify successful coastal MAR systems for the Netherlands (Amsterdam and The Hague areas), Spain (Barcelona (Llobregat) delta), Italy (Po delta), Belgium (De Panne), and Portugal (the Algarve). These include increasing artificial recharge in upland areas to enlarge the outflow of fresh groundwater through the coastal aquifer, injecting or infiltrating (purified) freshwater near the shoreline to create freshwater injection barriers, and enabling land reclamation to create a foreland where a freshwater body can develop or delay the inflow of saline groundwater. This is, for instance, a consequence of the sand suppletion (the Sand Engine in South Holland, the Netherlands), where the foreland better protects the low-lying hinterland against flooding (Huizer et al., 2016).
6.4 Receptor and consequences of saltwater intrusion
The progressive salinization of water resources has severe and long-lasting consequences for several social (e.g., reduction in the drinking water reservoirs), economic (e.g., water systems, agricultural production, and land losses), and environmental (e.g., degradation or losses of freshwater habitats and changes in biodiversity) issues. According to Cooper et al. (1964), groundwater SWI is a natural occurrence in coastal groundwater systems. However, human activities such as excessive freshwater pumping from coastal aquifers (Custodio, 2002; Custodio and Bruggeman, 1987; Mastrocicco and Colombani, 2021; Schmork and Mercado, 1969) often disrupt this natural process and even cause land subsidence (Minderhoud et al., 2017). The interaction between groundwater and surface water can also be significant, with saline groundwater sometimes exfiltrating towards surface water systems (de Louw et al., 2011; Delsman, 2015) and negatively impacting agricultural use and nature (Stofberg et al., 2015).
In densely populated and industrialized coastal regions around the world (Neumann et al., 2015), including areas in Europe, groundwater often serves as the primary source of freshwater. Several studies have examined the future availability of groundwater worldwide under climate change and related SLR (Green et al., 2011; Taylor et al., 2013) and human activities (e.g., Wada et al., 2010, 2014). Projected SLR is expected to exacerbate water stress in these densely populated areas, potentially leading to the overexploitation and salinization of groundwater resources due to upconing (the upward flow of) saline water. This situation is further compounded by the growing demand for freshwater in the future. Groundwater extraction in deltas leads to the accelerated salinization of coastal freshwater aquifers and land subsidence if groundwater infiltration is limited by low permeable layers (Herrera-García et al., 2021).
The influence of SLR on coastal groundwater systems has been studied since the 1990s (Navoy, 1991; Oude Essink, 1996; Sherif and Singh, 1999). More recent contributions concern (global) conceptual analyses based on analytical comparisons of freshwater–saltwater interfaces (Chang et al., 2011; Chesnaux, 2015; Chesnaux et al., 2021; Ferguson and Gleeson, 2012; Mazi et al., 2014; Werner and Simmons, 2009), 2D cross-sectional model studies (Ketabchi and Jahangir, 2021; Michael et al., 2013; Zamrsky et al., 2024), and 3D model studies (Befus et al., 2020; Loáiciga, 2009; Mabrouk et al., 2018; Masterson and Garabedian, 2007; Oude Essink et al., 2010; Rasmussen et al., 2013; Vandenbohede et al., 2008; Delsman et al., 2023), whereas the effects of SLR on coastal groundwater systems have been reviewed in Ketabchi et al. (2016).
In this chapter, the main impacts of SLR, namely coastal flooding, erosion, and saltwater intrusion, have been reviewed using the concept of the source, pathway, and receptor. Regarding coastal flooding, SLR, along with changes in storm surge and wave height and, in some places, increases in tidal range, has driven an increase in the frequency with which extreme coastal water levels are exceeding high thresholds (source). This, in turn, along with an ongoing decline in the extent of natural habitats that act as a buffer against flooding (pathways) and rapid population growth and urban encroachment in flood-prone areas (receptors), has driven an increase in coastal flooding and its impact (consequences) around the coast of Europe. However, current flood risk around the coastline of Europe would be considerably higher without the decades of investment into extensive flood risk management infrastructure and advances in flood forecasting and emergency response. At the same time, losses in major events that exceed defense design standards are growing and are expected to increase massively in the future with higher rates of SLR, unless further adaptation is taken. Furthermore, events with low flood levels (i.e., nuisance flooding) are likely to increase, causing widespread disruption of everyday routine activities and property damages, especially in low-lying areas.
Coastal erosion shares the same source as flooding, i.e., extreme waves and storm surge, even though in many areas additional long-term anthropogenic, geological, and climatic factors are also important, as they affect sediment budgets (Pathways). A total of more than 8200 km of Europe's sandy beaches has significantly retreated over the last decades, as found at various locations across the continent (receptors). Climate change and rising seas are expected to accelerate the current trend, and retreating shorelines combined with built areas backshore will result in coastal squeeze and threaten sandy beaches. The above will not only have social, economic, and ecological consequences but is also expected to also exacerbate coastal flooding.
In many regions in Europe, saltwater intrusion into surface and groundwater systems is emerging as a problem. Sea level rise is an important driver (source). SLR facilitates coastal inundation and the intrusion of marine waters into freshwater resources (pathway). But other (human) processes also play an important role, such as groundwater extractions and low-lying areas attracting saltwater and reduced fresh river flows. These processes diminish freshwater availability, leading to health risks caused by drinking water that is too salty and less freshwater for economic purposes (e.g., salt damage to crops) and nature (stresses on existing biodiversity). A wide variety of adaptation measurements has been developed over the last few decades, reducing the impact of saltwater intrusion. At the same time, projected SLR is expected to increase the impact of saltwater intrusion in the densely populated coastal areas of Europe, particularly in combination with the growing demand for freshwater in the future. The first signals of the compounding effects of flooding, erosion, and saltwater intrusion are emerging. The adaptation measures also reduced exposure at some places, but an overview on these is lacking. This is also true for the possible negative impact of SLR on coastal ecosystems and estuaries (e.g., degradation of marshes, wetlands, and saltwater intrusions). This impact maybe through SLR itself or indirectly through measures implemented to protect the land from flooding or other adverse effects, but there is too little scientific literature available to assess this.
In summary, we can conclude that the impacts of SLR are emerging at many places across Europe, and it might be expected that these impacts on freshwater availability will increase over time, thereby providing an incentive for further mitigation measures, whereas, at the same time, smart adaptation measures are needed to reduce the impacts themselves.
No data sets were used in this article.
RvdW and AM coordinated the paper. RvdW led the writing of the abstract. Section 2 was led by PL. IDH and RvdW drafted Sect. 3. IDH and AT drafted Sect. 4. MV, PC, and AL drafted Sect. 5. GOE, CF, and DB drafted Sect. 6. All authors contributed to the different versions of the entire paper.
The contact author has declared that none of the authors has any competing interests.
Publisher's note: Copernicus Publications remains neutral with regard to jurisdictional claims made in the text, published maps, institutional affiliations, or any other geographical representation in this paper. While Copernicus Publications makes every effort to include appropriate place names, the final responsibility lies with the authors.
Kate Davis from Southampton University drafted Figs. 2, 3, 7, and 10. Dominik Paprotny drafted Fig. 6. Panos Athanasiou drafted Fig. 9.
This paper is part of the 1st Assessment Report of the Knowledge Hub on Sea Level Rise (https://knowledgehubsealevelrise.org/, last access: 25 September 2024), a joint initiative by the Joint Programming Initiatives for Connecting Climate Knowledge for Europe (https://jpi-climate.eu/, last access: 25 September 2024) and Healthy and Productive Seas and Oceans (https://jpi-oceans.eu/en, last access: 25 September 2024), and is endorsed as a UN Ocean Decade project. This assessment report has been made possible through the dedicated efforts of the knowledge hub experts, authors, editors, reviewers, and contributors to scoping workshops and the Sea Level Rise Conference 2022 held in Venice in October 2022.
This research has been supported by the Ministerio de Ciencia e Innovación (MCIN/AEI and NextGenerationEU/PRTR) through the Ramón y Cajal program (grant no. RYC2021-030873-I); the UK Natural Environment Research Council grant CHANCE (grant no. NE/S010262/1); CoCliCo, which has received funding from the European Union's Horizon 2020 research and innovation program (grant no. 101003598); and the NPP.
This paper was edited by Nadia Pinardi and reviewed by Goneri Le Cozannet and Claudia Romagnoli.
Aarnes, O., Reistad, M., Breivik, O., Bitner-Gregersen, E., Eide, L. I., Gramstad, O., Magnusson, A. K., Natvig, B., and Vanem, E.: Projected changes in significant wave height towards the end of the 21st century – Northeast Atlantic, J. Geophys. Res.-Oceans, 122, 3394–3403, 2017.
Abadie, L. M., Jackson, L. P., Sainz de Murieta, E., Jevrejeva, S., and Galarraga, I.: Comparing urban coastal flood risk in 136 cities under two alternative sea-level projections: RCP 8.5 and an expert opinion-based high-end scenario, Ocean Coast. Manage., 193, 105249, https://doi.org/10.1016/j.ocecoaman.2020.105249, 2020.
Ali, E., Cramer, W., Carnicer, J., Georgopoulou, E., Hilmi, N., Le Cozannet, G., and Lionello, P.: Cross-Chapter Paper 4: Mediterranean Region, in: Climate Change 2022: Impacts, Adaptation and Vulnerability. Contribution of Working Group II to the Sixth Assessment Report of the Intergovernmental Panel on Climate Change, edited by: Pörtner, H.-O., Roberts, C. D., Tignor, M., Poloczanska, E., Mintenbeck, K., Alegria, A., Craig, M., Langsdorf, S., Löschke, S., Möller, V., Okem, A., and Rama, B., Cambridge, UK and New York, NY, USA, 2233–2272, https://doi.org/10.1017/9781009325844.021, 2022.
Alvarez-Cuesta, M., Toimil, A., and Losada, I. J.: Modelling long-term shoreline evolution in highly anthropized coastal areas. Part 2: Assessing the response to climate change, Coast. Eng., 168, 103961, https://doi.org/10.1016/j.coastaleng.2021.103961, 2021.
Andreadis, K. M., Wing, O. E. J., Colven, E., Gleason, C. J., Bates, P. D., and Brown, C. M.: Urbanizing the floodplain: global changes of imperviousness in flood-prone areas, Environ. Res. Lett., 17, 104024, https://doi.org/10.1088/1748-9326/ac9197, 2022.
Anthony, E. J., Almar, R., Besset, M., Reyns, J., Laibi, R., Ranasinghe, R., Abessolo Ondoa, G., and Vacchi, M.: Response of the Bight of Benin (Gulf of Guinea, West Africa) coastline to anthropogenic and natural forcing, Part 2: Sources and patterns of sediment supply, sediment cells, and recent shoreline change, Cont. Shelf Res., 173, 93–103, https://doi.org/10.1016/j.csr.2018.12.006, 2019.
Armanuos, A. M., Al-Ansari, N., and Yaseen, Z. M.: Underground Barrier Wall Evaluation for Controlling Saltwater Intrusion in Sloping Unconfined Coastal Aquifers, Water, 12, 2403, https://doi.org/10.3390/w12092403, 2020.
Arns, A., Dangendorf, S., Jensen, J., Talke, S., Bender, J., and Pattiaratchi, C.: Sea-level rise induced amplification of coastal protection design heights, Sci. Rep., 7, 40171, https://doi.org/10.1038/srep40171, 2017.
Arns, A., Wahl, T., Wolff, C., Vafeidis, A. T., Haigh, I. D., Woodworth, P., Niehüser, S., and Jensen, J.: Non-linear interaction modulates global extreme sea levels, coastal flood exposure, and impacts, Nat. Commun., 11, 1918, https://doi.org/10.1038/s41467-020-15752-5, 2020.
Athanasiou, P., van Dongeren, A., Giardino, A., Vousdoukas, M. I., Ranasinghe, R., and Kwadijk, J.: Uncertainties in projections of sandy beach erosion due to sea level rise: an analysis at the European scale, Sci. Rep., 10, 11895, https://doi.org/10.1038/s41598-020-68576-0, 2020.
Barnard, P. L., Short, A. D., Harley, M. D., Splinter, K. D., Vitousek, S., Turner, I. L., Allan, J., Banno, M., Bryan, K. R., Doria, A., Hansen, J. E., Kato, S., Kuriyama, Y., Randall-Goodwin, E., Ruggiero, P., Walker, I. J., and Heathfield, D. K.: Coastal vulnerability across the Pacific dominated by El Niño/Southern Oscillation, Nat. Geosci., 8, 801–807, https://doi.org/10.1038/ngeo2539, 2015.
Barnard, P. L., Erikson, L. H., Foxgrover, A. C., Hart, J. A. F., Limber, P., O'Neill, A. C., van Ormondt, M., Vitousek, S., Wood, N., Hayden, M. K., and Jones, J. M.: Dynamic flood modeling essential to assess the coastal impacts of climate change, Sci. Rep., 9, 4309, https://doi.org/10.1038/s41598-019-40742-z, 2019.
Bednar-Friedl, Biesbroek, R., Schmidt, D. N., Alexander, P., Børsheim, K. Y., Carnicer, J., Georgopoulou, E., Haasnoot, M., Le Cozannet, G., Lionello, P., Lipka, O., Möllmann, C., Muccione, V., Mustonen, T., Piepenburg, D., and Whitmarsh, L.: Chapter 13 – Europe, in: Climate Change 2022: Impacts, Adaptation and Vulnerability. Contribution of Working Group II to the Sixth Assessment Report of the Intergovernmental Panel on Climate Change, edited by: Pörtner, H.-O., Roberts, D. C., Tignor, M., Poloczanska, E. S., Mintenbeck, K., Alegría, A., Craig, M., Langsdorf, S., Löschke, S., Möller, V., Okem, A., and Rama, B., Cambridge University Press, Cambridge, https://doi.org/10.1017/9781009325844.015, 2022.
Befus, K. M., Barnard, P. L., Hoover, D. J., Finzi Hart, J. A., and Voss, C. I.: Increasing threat of coastal groundwater hazards from sea-level rise in California, Nat. Clim. Chang., 10, 946–952, https://doi.org/10.1038/s41558-020-0874-1, 2020.
Bellafiore, D., Ferrarin, C., Maicu, F., Manfè, G., Lorenzetti, G., Umgiesser, G., Zaggia, L., and Levinson, A. V.: Saltwater Intrusion in a Mediterranean Delta Under a Changing Climate, J. Geophys. Res.-Oceans, 126, e2020JC016437, https://doi.org/10.1029/2020JC016437, 2021.
Benetazzo, A., Davison, S., Barbariol, F., Mercogliano, P., Favaretto, C., and Sclavo, M.: Correction of ERA5 Wind for Regional Climate Projections of Sea Waves, Water, 14, 1590, https://doi.org/10.3390/w14101590, 2022.
Bermúdez, M., Cea, L., and Sopelana, J.: Quantifying the role of individual flood drivers and their correlations in flooding of coastal river reaches, Stoch. Env. Res. Risk A., 33, 1851–1861, https://doi.org/10.1007/s00477-019-01733-8, 2019.
Bermúdez, M., Farfán, J. F., Willems, P., and Cea, L.: Assessing the Effects of Climate Change on Compound Flooding in Coastal River Areas, Water Resour. Res., 57, e2020WR029321, https://doi.org/10.1029/2020WR029321, 2021.
Bertels, D. and Willems, P.: Climate change impact on salinization of drinking water inlets along the Campine Canals, Belgium, Journal of Hydrology: Regional Studies, 42, 101129, https://doi.org/10.1016/j.ejrh.2022.101129, 2022.
Bevacqua, E., Maraun, D., Hobæk Haff, I., Widmann, M., and Vrac, M.: Multivariate statistical modelling of compound events via pair-copula constructions: analysis of floods in Ravenna (Italy), Hydrol. Earth Syst. Sci., 21, 2701–2723, https://doi.org/10.5194/hess-21-2701-2017, 2017.
Bevacqua, E., Maraun, D., Vousdoukas, M. I., Voukouvalas, E., Vrac, M., Mentaschi, L., and Widmann, M.: Higher probability of compound flooding from precipitation and storm surge in Europe under anthropogenic climate change, Science Advances, 5, eaaw5531, https://doi.org/10.1126/sciadv.aaw5531, 2019.
Bevacqua, E., Vousdoukas, M. I., Shepherd, T. G., and Vrac, M.: Brief communication: The role of using precipitation or river discharge data when assessing global coastal compound flooding, Nat. Hazards Earth Syst. Sci., 20, 1765–1782, https://doi.org/10.5194/nhess-20-1765-2020, 2020a.
Bevacqua, E., Vousdoukas, M. I., Zappa, G., Hodges, K., Shepherd, T. G., Maraun, D., Mentaschi, L., and Feyen, L.: More meteorological events that drive compound coastal flooding are projected under climate change, Communications Earth & Environment, 1, 47, https://doi.org/10.1038/s43247-020-00044-z, 2020b.
Bevacqua, E., De Michele, C., Manning, C., Couasnon, A., Ribeiro, A. F. S., Ramos, A. M., Vignotto, E., Bastos, A., Blesić, S., Durante, F., Hillier, J., Oliveira, S. C., Pinto, J. G., Ragno, E., Rivoire, P., Saunders, K., Wiel, K., Wu, W., Zhang, T., and Zscheischler, J.: Guidelines for Studying Diverse Types of Compound Weather and Climate Events, Earths Future, 9, e2021EF002340, https://doi.org/10.1029/2021EF002340, 2021.
Bilskie, M. V., Hagen, S. C., Medeiros, S. C., and Passeri, D. L.: Dynamics of sea level rise and coastal flooding on a changing landscape, Geophys. Res. Lett., 41, 927–934, https://doi.org/10.1002/2013GL058759, 2014.
Bisaro, A., Galluccio, G., Fiorini Beckhauser, E., Biddau, F., David, R., d'Hont, F., Góngora Zurro, A., Le Cozannet, G., McEvoy, S., Pérez Gómez, B., Romagnoli, C., Sini, E., and Slinger, J.: Sea Level Rise in Europe: Governance context and challenges, in: Sea Level Rise in Europe: 1st Assessment Report of the Knowledge Hub on Sea Level Rise (SLRE1), edited by: van den Hurk, B., Pinardi, N., Kiefer, T., Larkin, K., Manderscheid, P., and Richter, K., Copernicus Publications, State Planet, 3-slre1, 7, https://doi.org/10.5194/sp-3-slre1-7-2024, 2024.
Bonaldo, D., Bellafiore, D., Ferrarin, C., Ferretti, R., Ricchi, A., Sangelantoni, L., and Vitelletti, M. L.: The summer 2022 drought: a taste of future climate for the Po valley (Italy)?, Reg. Environ. Change, 23, 1, https://doi.org/10.1007/s10113-022-02004-z, 2023.
Bonte, M. and Zwolsman, J. J. G.: Climate change induced salinisation of artificial lakes in the Netherlands and consequences for drinking water production, Water Res., 44, 4411–4424, https://doi.org/10.1016/j.watres.2010.06.004, 2010.
Bosserelle, C., Gallop, S. L., Haigh, I. D., and Pattiaratchi, C. B.: The Influence of Reef Topography on Storm-Driven Sand Flux, J. Mar. Sci. Eng., 9, 272, https://doi.org/10.3390/jmse9030272, 2021.
Boucharel, J., David, M., Almar, R., and Melet, A.: Contrasted influence of climate modes teleconnections to the interannual variability of coastal sea level components–implications for statistical forecasts, Clim. Dynam., 61, 4011–4023, https://doi.org/10.1007/s00382-023-06771-1, 2023.
Bove, G., Becker, A., Sweeney, B., Vousdoukas, M., and Kulp, S.: A method for regional estimation of climate change exposure of coastal infrastructure: Case of USVI and the influence of digital elevation models on assessments, Sci. Total Environ., 710, 136162, https://doi.org/10.1016/j.scitotenv.2019.136162, 2020.
Brand, E., Ramaekers, G., and Lodder, Q.: Dutch experience with sand nourishments for dynamic coastline conservation – An operational overview, Ocean Coast. Manage., 217, 106008, https://doi.org/10.1016/j.ocecoaman.2021.106008, 2022.
Breili, K., Simpson, M. J. R., Klokkervold, E., and Roaldsdotter Ravndal, O.: High-accuracy coastal flood mapping for Norway using lidar data, Nat. Hazards Earth Syst. Sci., 20, 673–694, https://doi.org/10.5194/nhess-20-673-2020, 2020.
Bruun, P.: Sea-level rise as a cause of shore erosion, Journal of the Waterways and Harbors division, 88, 117–130, 1962.
Calafat, F. M. and Marcos, M.: Probabilistic reanalysis of storm surge extremes in Europe, P. Natl. Acad. Sci. USA, 117, 1877–1883, https://doi.org/10.1073/pnas.1913049117, 2020.
Calafat, F. M., Wahl, T., Tadesse, M. G., and Sparrow, S. N.: Trends in Europe storm surge extremes match the rate of sea-level rise, Nature, 603, 841–845, https://doi.org/10.1038/s41586-022-04426-5, 2022.
Campbell, D. and Keddy, P.: The Roles of Competition and Facilitation in Producing Zonation Along an Experimental Flooding Gradient: a Tale of Two Tails with Ten Freshwater Marsh Plants, Wetlands, 42, 5, https://doi.org/10.1007/s13157-021-01524-4, 2022.
Camus, P., Haigh, I. D., Nasr, A. A., Wahl, T., Darby, S. E., and Nicholls, R. J.: Regional analysis of multivariate compound coastal flooding potential around Europe and environs: sensitivity analysis and spatial patterns, Nat. Hazards Earth Syst. Sci., 21, 2021–2040, https://doi.org/10.5194/nhess-21-2021-2021, 2021.
Camus, P., Haigh, I. D., Wahl, T., Nasr, A. A., Méndez, F. J., Darby, S. E., and Nicholls, R. J.: Daily synoptic conditions associated with occurrences of compound events in estuaries along North Atlantic coastlines, Int. J. Climatol., 42, 5694–5713, https://doi.org/10.1002/joc.7556, 2022.
Cantelon, J. A., Guimond, J. A., Robinson, C. E., Michael, H. A., and Kurylyk, B. L.: Vertical Saltwater Intrusion in Coastal Aquifers Driven by Episodic Flooding: A Review, Water Resour. Res., 58, e2022WR032614, https://doi.org/10.1029/2022WR032614, 2022.
Carbognin, L. and Tosi, L.: Interaction between Climate Changes, Eustacy and Land Subsidence in the North Adriatic Region, Italy, Mar. Ecol., 23, 38–50, https://doi.org/10.1111/j.1439-0485.2002.tb00006.x, 2002.
Cardona, O. D., Van Aalst, M. K., Birkmann, J., Fordham, M., Mc Gregor, G., Rosa, P., Pulwarty, R. S., Schipper, E. L. F., Sinh, B. T., Décamps, H., Keim, M., Davis, I., Ebi, K. L., Lavell, A., Mechler, R., Murray, V., Pelling, M., Pohl, J., Smith, A. O., and Thomalla, F.: Determinants of risk: Exposure and vulnerability, in: Managing the Risks of Extreme Events and Disasters to Advance Climate Change Adaptation: Special Report of the Intergovernmental Panel on Climate Change, edited by: Field, C. B., Barros, V. R., Stocker, T. F., Qin, D., Dokken, D. J., Ebi, K. L., Mastrandrea, M. D., Mach, K. J., Plattner, G.-K., Allen, S. K., Tignor, M., and Midgley, P. M., Cambridge University Press, 65–108, https://doi.org/10.1017/CBO9781139177245.005, 2012.
Cazenave, A. and Le Cozannet, G.: Sea level rise and its coastal impacts, Earths Future, 2, 15–34, 2014.
Chaigneau, A. A., Law-Chune, S., Melet, A., Voldoire, A., Reffray, G., and Aouf, L.: Impact of sea level changes on future wave conditions along the coasts of western Europe, Ocean Sci., 19, 1123–1143, https://doi.org/10.5194/os-19-1123-2023, 2023.
Chang, S. W., Clement, T. P., Simpson, M. J., and Lee, K.-K.: Does sea-level rise have an impact on saltwater intrusion?, Adv. Water Resour., 34, 1283–1291, https://doi.org/10.1016/j.advwatres.2011.06.006, 2011.
Chapapría, V. E., Peris, J. S., and González-Escrivá, J. A.: Coastal Monitoring Using Unmanned Aerial Vehicles (UAVs) for the Management of the Spanish Mediterranean Coast: The Case of Almenara-Sagunto, Int. J. Env. Res. Pub. He., 19, 5457, https://doi.org/10.3390/ijerph19095457, 2022.
Chesnaux, R.: Closed-form analytical solutions for assessing the consequences of sea-level rise on groundwater resources in sloping coastal aquifers, Hydrogeol. J., 23, 1399–1413, https://doi.org/10.1007/s10040-015-1276-8, 2015.
Chesnaux, R., Marion, D., Boumaiza, L., Richard, S., and Walter, J.: An analytical methodology to estimate the changes in fresh groundwater resources with sea-level rise and coastal erosion in strip-island unconfined aquifers: illustration with Savary Island, Canada, Hydrogeol. J., 29, 1355–1364, https://doi.org/10.1007/s10040-020-02300-0, 2021.
Coco, G., Senechal, N., Rejas, A., Bryan, K. R., Capo, S., Parisot, J. P., Brown, J. A., and MacMahan, J. H. M.: Beach response to a sequence of extreme storms, Geomorphology, 204, 493–501, https://doi.org/10.1016/j.geomorph.2013.08.028, 2014.
Cooley, S., Schoeman, D., Bopp, L., Boyd, P., Donner, S., Ghebrehiwet, D. Y., Ito, S.-I., Kiessling, W., Martinetto, P., Ojea, E., Racault, M.-F., Rost, B., and Skern-Mauritzen, M.: Oceans and Coastal Ecosystems and Their Services, in: Climate Change 2022: Impacts, Adaptation and Vulnerability. Contribution of Working Group II to the Sixth Assessment Report of the Intergovernmental Panel on Climate Change, edited by: Pörtner, H.-O., Roberts, D. C., Tignor, M., Poloczanska, E. S., Mintenbeck, K., Alegría, A., Craig, M., Langsdorf, S., Löschke, S., Möller, V., Okem, A., and Rama, B., Cambridge University Press, Cambridge, UK and New York, NY, USA, 379–550, https://doi.org/10.1017/9781009325844.005, 2022.
Cooper, H. H. J., Kohout, F. A., Henry, H. R., and Glover, R. E.: Sea Water in Coastal Aquifers, US Department of the interior, Geological Survey water supply paper 1613-C, Washington, 1964.
Core Writing Team, Lee, H., and Romero, J. (Eds.): IPCC, 2023: Climate Change 2023: Synthesis Report. Contribution of Working Groups I, II and III to the Sixth Assessment Report of the Intergovernmental Panel on Climate Change, Intergovernmental Panel on Climate Change (IPCC), Geneva, Switzerland, https://doi.org/10.59327/IPCC/AR6-9789291691647, 2023.
Couasnon, A., Eilander, D., Muis, S., Veldkamp, T. I. E., Haigh, I. D., Wahl, T., Winsemius, H. C., and Ward, P. J.: Measuring compound flood potential from river discharge and storm surge extremes at the global scale, Nat. Hazards Earth Syst. Sci., 20, 489–504, https://doi.org/10.5194/nhess-20-489-2020, 2020.
Custodio, E.: Aquifer overexploitation: what does it mean?, Hydrogeol. J., 10, 254–277, https://doi.org/10.1007/s10040-002-0188-6, 2002.
Custodio, E. and Bruggeman, G. A.: Groundwater problems in coastal areas: a contribution to the International Hydrological Programme, Unesco, Paris, 596 pp., ISBN 978-92-3-102415-3, 1987.
D'Anna, M., Castelle, B., Idier, D., Rohmer, J., Le Cozannet, G., Thieblemont, R., and Bricheno, L.: Uncertainties in Shoreline Projections to 2100 at Truc Vert Beach (France): Role of Sea-Level Rise and Equilibrium Model Assumptions, J. Geophys. Res.-Earth, 126, e2021JF006160, https://doi.org/10.1029/2021JF006160, 2021.
D'Anna, M., Idier, D., Castelle, B., Rohmer, J., Cagigal, L., and Mendez, F. J.: Effects of stochastic wave forcing on probabilistic equilibrium shoreline response across the 21st century including sea-level rise, Coast. Eng., 175, 104149, https://doi.org/10.1016/j.coastaleng.2022.104149, 2022.
Davenport, J. and Davenport, J. L.: The impact of tourism and personal leisure transport on coastal environments: A review, Estuar. Coast. Shelf S., 67, 280–292, https://doi.org/10.1016/j.ecss.2005.11.026, 2006.
Dawson, D., Shaw, J., and Roland Gehrels, W.: Sea-level rise impacts on transport infrastructure: The notorious case of the coastal railway line at Dawlish, England, J. Transp. Geogr., 51, 97–109, https://doi.org/10.1016/j.jtrangeo.2015.11.009, 2016.
Dawson, R. J., Dickson, M. E., Nicholls, R. J., Hall, J. W., Walkden, M. J. A., Stansby, P. K., Mokrech, M., Richards, J., Zhou, J., Milligan, J., Jordan, A., Pearson, S., Rees, J., Bates, P. D., Koukoulas, S., and Watkinson, A. R.: Integrated analysis of risks of coastal flooding and cliff erosion under scenarios of long term change, Climatic Change, 95, 249–288, https://doi.org/10.1007/s10584-008-9532-8, 2009.
de Louw, P. G. B., Eeman, S., Siemon, B., Voortman, B. R., Gunnink, J., van Baaren, E. S., and Oude Essink, G. H. P.: Shallow rainwater lenses in deltaic areas with saline seepage, Hydrol. Earth Syst. Sci., 15, 3659–3678, https://doi.org/10.5194/hess-15-3659-2011, 2011.
Delsman, J. R.: Saline groundwater – surface water interaction in coastal lowlands, IOS Press BV, Amsterdam, 185 pp., https://doi.org/10.3233/978-1-61499-518-0-i, 2015.
Delsman, J. R., Van Baaren, E. S., Siemon, B., Dabekaussen, W., Karaoulis, M. C., Pauw, P. S., Vermaas, T., Bootsma, H., De Louw, P. G. B., Gunnink, J. L., Dubelaar, C. W., Menkovic, A., Steuer, A., Meyer, U., Revil, A., and Oude Essink, G. H. P.: Large-scale, probabilistic salinity mapping using airborne electromagnetics for groundwater management in Zeeland, the Netherlands, Environ. Res. Lett., 13, 084011, https://doi.org/10.1088/1748-9326/aad19e, 2018.
Delsman, J. R., Mulder, T., Romero Verastegui, B., Bootsma, H., Zitman, P., Huizer, S., and Oude Essink, G. H. P.: Reproducible construction of a high-resolution national variable-density groundwater salinity model for the Netherlands, Environ. Model. Softw., 164, 105683, https://doi.org/10.1016/j.envsoft.2023.105683, 2023.
de Santiago, I., Camus, P., González, M., Liria, P., Epelde, I., Chust, G., del Campo, A., and Uriarte, A.: Impact of climate change on beach erosion in the Basque Coast (NE Spain), Coast. Eng., 167, 103916, https://doi.org/10.1016/j.coastaleng.2021.103916, 2021.
Didier, D., Bernatchez, P., Boucher-Brossard, G., Lambert, A., Fraser, C., Barnett, R., and Van-Wierts, S.: Coastal Flood Assessment Based on Field Debris Measurements and Wave Runup Empirical Model, J. Mar. Sci. Eng., 3, 560–590, https://doi.org/10.3390/jmse3030560, 2015.
Dierberg, F. E. and Kiattisimkul, W.: Issues, impacts, and implications of shrimp aquaculture in Thailand, Environ. Manage., 20, 649–666, https://doi.org/10.1007/BF01204137, 1996.
Dillon, P., Stuyfzand, P., Grischek, T., Lluria, M., Pyne, R. D. G., Jain, R. C., Bear, J., Schwarz, J., Wang, W., Fernandez, E., Stefan, C., Pettenati, M., Van Der Gun, J., Sprenger, C., Massmann, G., Scanlon, B. R., Xanke, J., Jokela, P., Zheng, Y., Rossetto, R., Shamrukh, M., Pavelic, P., Murray, E., Ross, A., Bonilla Valverde, J. P., Palma Nava, A., Ansems, N., Posavec, K., Ha, K., Martin, R., and Sapiano, M.: Sixty years of global progress in managed aquifer recharge, Hydrogeol. J., 27, 1–30, https://doi.org/10.1007/s10040-018-1841-z, 2019.
Dodet, G., Melet, A., Ardhuin, F., Bertin, X., Idier, D., and Almar, R.: The Contribution of Wind-Generated Waves to Coastal Sea-Level Changes, Surv. Geophys., 40, 1563–1601, https://doi.org/10.1007/s10712-019-09557-5, 2019.
Dodman, D., Hayward, B., Pelling, M., Castan Broto, V., Chow, W., Chu, E., Dawson, R., Khirfan, L., McPhearson, T., Prakash, A., Zheng, Y., and Ziervogel, G.: Cities, Settlements, and Key Infrastructure, in: Climate Change 2022: Impacts, Adaptation and Vulnerability. Contribution of Working Group II to the Sixth Assessment Report of the Intergovernmental Panel on Climate Change, edited by: Pörtner, H.-O., Roberts, C. D., Tignor, M., Poloczanska, E., Mintenbeck, K., Alegria, A., Craig, M., Langsdorf, S., Löschke, S., Möller, V., Okem, A., and Rama, B., Cambridge University Press, Cambridge, UK and New York, NY, USA, 2233–2272, https://doi.org/10.1017/9781009325844.021, 2022.
Donovan, B., Horsburgh, K., Ball, T., and Westbrook, G.: Impacts of climate change on coastal flooding, MCCIP Sci. Rev., 2013, 211–218, https://doi.org/10.14465/2013.ARC22.211-218, 2013.
Duque, C., Meyer, R., and Sonnenborg, T. O.: Saltwater intrusion in Denmark, Boletín Geológico Y Minero, 133, 29–46, https://doi.org/10.21701/bolgeomin/133.1/002, 2022.
Eijgenraam, C., Kind, J., Bak, C., Brekelmans, R., den Hertog, D., Duits, M., Roos, K., Vermeer, P., and Kuijken, W.: Economically Efficient Standards to Protect the Netherlands Against Flooding, Interfaces, 44, 7–21, https://doi.org/10.1287/inte.2013.0721, 2014.
Eilander, D., Couasnon, A., Ikeuchi, H., Muis, S., Yamazaki, D., Winsemius, H. C., and Ward, P. J.: The effect of surge on riverine flood hazard and impact in deltas globally, Environ. Res. Lett., 15, 104007, https://doi.org/10.1088/1748-9326/ab8ca6, 2020.
Environment Agency: Thames Estuary 2100: 10-Year Review – Technical Monitoring Report, Environment Agency, https://www.gov.uk/government/publications/thames-estuary-2100-te2100-monitoring-reviews/thames-estuary-2100-10-year-monitoring-review-2021#conclusion (last access: 14 July 2024), 2021.
European Commission: Towards an EU research and innovation policy agenda for nature-based solutions & re-naturing cities – Final report of the Horizon 2020 expert group on “Nature-based solutions and re-naturing cities”, European Commission, https://doi.org/10.2777/479582, 2015.
EUROSION: Living with coastal erosion in Europe: Sediment and Space for Sustainability. PART II: Maps and Statistics. Final Report of the Project “Coastal erosion – Evaluation of the need for action”, Directorate General Environment, European Commission, 2004.
Feagin, R. A., Furman, M., Salgado, K., Martinez, M. L., Innocenti, R. A., Eubanks, K., Figlus, J., Huff, T. P., Sigren, J., and Silva, R.: The role of beach and sand dune vegetation in mediating wave run up erosion, Estuar. Coast. Shelf S., 219, 97–106, https://doi.org/10.1016/j.ecss.2019.01.018, 2019.
Ferguson, G. and Gleeson, T.: Vulnerability of coastal aquifers to groundwater use and climate change, Nat. Clim. Change, 2, 342–345, https://doi.org/10.1038/nclimate1413, 2012.
Ferrarin, C., Tomasin, A., Bajo, M., Petrizzo, A., and Umgiesser, G.: Tidal changes in a heavily modified coastal wetland, Cont. Shelf Res., 101, 22–33, https://doi.org/10.1016/j.csr.2015.04.002, 2015.
Ferrarin, C., Lionello, P., Orlić, M., Raicich, F., and Salvadori, G.: Venice as a paradigm of coastal flooding under multiple compound drivers, Sci. Rep., 12, 5754, https://doi.org/10.1038/s41598-022-09652-5, 2022.
Ferreira, Ó., Kupfer, S., and Costas, S.: Implications of sea-level rise for overwash enhancement at South Portugal, Nat. Hazards, 109, 2221–2239, https://doi.org/10.1007/s11069-021-04917-0, 2021.
Gallop, S. L., Bosserelle, C., Pattiaratchi, C., and Eliot, I.: Rock topography causes spatial variation in the wave, current and beach response to sea breeze activity, Mar. Geol., 290, 29–40, https://doi.org/10.1016/j.margeo.2011.10.002, 2011.
Galluccio, G., Hinkel, J., Fiorini Beckhauser, E., Bisaro, A., Biancardi Aleu, R., Campostrini, P., Casas, M. F., Espin, O., and Vafeidis, A. T.: Sea Level Rise in Europe: Adaptation measures and decision-making principles, in: Sea Level Rise in Europe: 1st Assessment Report of the Knowledge Hub on Sea Level Rise (SLRE1), edited by: van den Hurk, B., Pinardi, N., Kiefer, T., Larkin, K., Manderscheid, P., and Richter, K., Copernicus Publications, State Planet, 3-slre1, 6, https://doi.org/10.5194/sp-3-slre1-6-2024, 2024.
Ganguli, P. and Merz, B.: Extreme Coastal Water Levels Exacerbate Fluvial Flood Hazards in Northwestern Europe, Sci. Rep., 9, 13165, https://doi.org/10.1038/s41598-019-49822-6, 2019a.
Ganguli, P. and Merz, B.: Trends in Compound Flooding in Northwestern Europe During 1901–2014, Geophys. Res. Lett., 46, 10810–10820, https://doi.org/10.1029/2019GL084220, 2019b.
Ganguli, P., Paprotny, D., Hasan, M., Güntner, A., and Merz, B.: Projected Changes in Compound Flood Hazard From Riverine and Coastal Floods in Northwestern Europe, Earths Future, 8, e2020EF001752, https://doi.org/10.1029/2020EF001752, 2020.
Gharagozlou, A., Dietrich, J. C., Karanci, A., Luettich, R. A., and Overton, M. F.: Storm-driven erosion and inundation of barrier islands from dune-to region-scales, Coast. Eng., 158, 103674, https://doi.org/10.1016/j.coastaleng.2020.103674, 2020.
Glavovic, B. C., Dawson, R., Chow, W., Garschagen, M., Haasnoot, M., Singh, C., and Thomas, A.: Cross-Chapter Paper 2: Cities and Settlements by the Sea, in: Climate Change 2022: Impacts, Adaptation and Vulnerability. Contribution of Working Group II to the Sixth Assessment Report of the Intergovernmental Panel on Climate Change, edited by: Pörtner, H.-O., Roberts, D. C., Tignor, M., Poloczanska, E. S., Mintenbeck, K., Alegría, A., Craig, M., Langsdorf, S., Löschke, S., Möller, V., Okem, A., and Rama, B., Cambridge University Press, Cambridge, UK and New York, NY, USA, 2163–2194, https://doi.org/10.1017/9781009325844.019, 2022.
Global Commission on Adaptation: Adapt Now: A Global Call for Leadership on Climate Resilience, World Resources Institute, Washington, DC, https://openknowledge.worldbank.org/handle/10986/32362 (last access: 14 July 2024), 2019.
Granger, C. W. J.: Estimating the probability of flooding on a tidal river, in: Essays in econometrics: Collected Papers of Clive W. J. Granger Volume 1, Spectral Analysis, Seasonality, Nonlinearity, Methodology, and Forecasting, Cambridge University Press, USA, 355–365, https://doi.org/10.1017/CBO9780511753961, 2001.
Grases, A., Gracia, V., García-León, M., Lin-Ye, J., and Sierra, J. P.: Coastal Flooding and Erosion under a Changing Climate: Implications at a Low-Lying Coast (Ebro Delta), Water, 12, 346, https://doi.org/10.3390/w12020346, 2020.
Green, T. R., Taniguchi, M., Kooi, H., Gurdak, J. J., Allen, D. M., Hiscock, K. M., Treidel, H., and Aureli, A.: Beneath the surface of global change: Impacts of climate change on groundwater, J. Hydrol., 405, 532–560, https://doi.org/10.1016/j.jhydrol.2011.05.002, 2011.
Grilli, A., Spaulding, M. L., Oakley, B. A., and Damon, C.: Mapping the coastal risk for the next century, including sea level rise and changes in the coastline: application to Charlestown RI, USA, Nat. Hazards, 88, 389–414, https://doi.org/10.1007/s11069-017-2871-x, 2017.
Haasnoot, M., Kwadijk, J., Alphen, J. van, Bars, D. L., Diermanse, F., Spek, A. van der, Essink, G. O., Delsman, J., and Mens, M.: Adaptation to uncertain sea-level rise; how uncertainty in Antarctic mass-loss impacts the coastal adaptation strategy of the Netherlands, Environ. Res. Lett., 15, 034007, https://doi.org/10.1088/1748-9326/ab666c, 2020.
Haigh, I. D., Eliot, M., and Pattiaratchi, C.: Global influences of the 18.61 year nodal cycle and 8.85 year cycle of lunar perigee on high tidal levels, J. Geophys. Res., 116, C06025, https://doi.org/10.1029/2010JC006645, 2011.
Haigh, I. D., Wadey, M. P., Gallop, S. L., Loehr, H., Nicholls, R. J., Horsburgh, K., Brown, J. M., and Bradshaw, E.: A user-friendly database of coastal flooding in the United Kingdom from 1915–2014, Scientific Data, 2, 150021, https://doi.org/10.1038/sdata.2015.21, 2015.
Haigh, I. D., Ozsoy, O., Wadey, M. P., Nicholls, R. J., Gallop, S. L., Wahl, T., and Brown, J. M.: An improved database of coastal flooding in the United Kingdom from 1915 to 2016, Scientific Data, 4, 170100, https://doi.org/10.1038/sdata.2017.100, 2017.
Haigh, I. D., Pickering, M. D., Green, J. A. M., Arbic, B. K., Arns, A., Dangendorf, S., Hill, D. F., Horsburgh, K., Howard, T., Idier, D., Jay, D. A., Jänicke, L., Lee, S. B., Müller, M., Schindelegger, M., Talke, S. A., Wilmes, S., and Woodworth, P. L.: The Tides They Are A-Changin': A Comprehensive Review of Past and Future Nonastronomical Changes in Tides, Their Driving Mechanisms, and Future Implications, Rev. Geophys., 58, e2018RG000636, https://doi.org/10.1029/2018RG000636, 2020.
Haigh, I. D., Nicholls, R. J., Penning-Rowsell, E. C., and Sayers, P.: Climate change impacts on coastal flooding relevant to the UK and Ireland, MCCIP Rolling Evidence Updates, MCCIP Science Review, 18 pp., https://doi.org/10.14465/2022.REU02.CFL, 2022.
Hall, A. E., Herbert, R. J. H., Britton, J. R., Boyd, I. M., and George, N. C.: Shelving the Coast With Vertipools: Retrofitting Artificial Rock Pools on Coastal Structures as Mitigation for Coastal Squeeze, Front. Mar. Sci., 6, 456, https://doi.org/10.3389/fmars.2019.00456, 2019.
Hallegatte, S., Green, C., Nicholls, R. J., and Corfee-Morlot, J.: Future flood losses in major coastal cities, Nat. Clim. Change, 3, 802–806, 2013.
Hansen, J. E. and Barnard, P. L.: Sub-weekly to interannual variability of a high-energy shoreline, Coast. Eng., 57, 959–972, https://doi.org/10.1016/j.coastaleng.2010.05.011, 2010.
Harley, M. D., Turner, I. L., Short, A. D., and Ranasinghe, R.: Assessment and integration of conventional, RTK-GPS and image-derived beach survey methods for daily to decadal coastal monitoring, Coast. Eng., 58, 194–205, https://doi.org/10.1016/j.coastaleng.2010.09.006, 2011.
He, F. J. and MacGregor, G. A.: Salt reduction lowers cardiovascular risk: meta-analysis of outcome trials, Lancet, 378, 380–382, https://doi.org/10.1016/S0140-6736(11)61174-4, 2011.
Heinrich, P., Hagemann, S., Weisse, R., and Gaslikova, L.: Changes in compound flood event frequency in northern and central Europe under climate change, Front. Clim., 5, 1227613, https://doi.org/10.3389/fclim.2023.1227613, 2023a.
Heinrich, P., Hagemann, S., Weisse, R., Schrum, C., Daewel, U., and Gaslikova, L.: Compound flood events: analysing the joint occurrence of extreme river discharge events and storm surges in northern and central Europe, Nat. Hazards Earth Syst. Sci., 23, 1967–1985, https://doi.org/10.5194/nhess-23-1967-2023, 2023b.
Hendry, A., Haigh, I. D., Nicholls, R. J., Winter, H., Neal, R., Wahl, T., Joly-Laugel, A., and Darby, S. E.: Assessing the characteristics and drivers of compound flooding events around the UK coast, Hydrol. Earth Syst. Sci., 23, 3117–3139, https://doi.org/10.5194/hess-23-3117-2019, 2019.
Herbert, E. R., Boon, P., Burgin, A. J., Neubauer, S. C., Franklin, R. B., Ardón, M., Hopfensperger, K. N., Lamers, L. P. M., and Gell, P.: A global perspective on wetland salinization: ecological consequences of a growing threat to freshwater wetlands, Ecosphere, 6, 1–43, https://doi.org/10.1890/ES14-00534.1, 2015.
Herrera-García, G., Ezquerro, P., Tomás, R., Béjar-Pizarro, M., López-Vinielles, J., Rossi, M., Mateos, R. M., Carreón-Freyre, D., Lambert, J., Teatini, P., Cabral-Cano, E., Erkens, G., Galloway, D., Hung, W.-C., Kakar, N., Sneed, M., Tosi, L., Wang, H., and Ye, S.: Mapping the global threat of land subsidence, Science, 371, 34–36, https://doi.org/10.1126/science.abb8549, 2021.
Hewitt, C., Mason, S., and Walland, D.: The Global Framework for Climate Services, Nat. Clim. Change, 2, 831–832, https://doi.org/10.1038/nclimate1745, 2012.
Hinkel, J., Nicholls, R. J., Tol, R. S. J., Wang, Z. B., Hamilton, J. M., Boot, G., Vafeidis, A. T., McFadden, L., Ganopolski, A., and Klein, R. J. T.: A global analysis of erosion of sandy beaches and sea-level rise: An application of DIVA, Global Planet. Change, 111, 150–158, https://doi.org/10.1016/j.gloplacha.2013.09.002, 2013.
Hinkel, J., Feyen, L., Hemer, M., Le Cozannet, G., Lincke, D., Marcos, M., Mentaschi, L., Merkens, J. L., de Moel, H., Muis, S., Nicholls, R. J., Vafeidis, A. T., van de Wal, R. S. W., Vousdoukas, M. I., Wahl, T., Ward, P. J., and Wolff, C.: Uncertainty and Bias in Global to Regional Scale Assessments of Current and Future Coastal Flood Risk, Earths Future, 9, e2020EF001882, https://doi.org/10.1029/2020EF001882, 2021.
Holman, R. A. and Stanley, J.: The history and technical capabilities of Argus, Coast. Eng., 54, 477–491, https://doi.org/10.1016/j.coastaleng.2007.01.003, 2007.
Horner-Devine, A. R., Hetland, R. D., and MacDonald, D. G.: Mixing and Transport in Coastal River Plumes, Annu. Rev. Fluid Mech., 47, 569–594, https://doi.org/10.1146/annurev-fluid-010313-141408, 2015.
Horsburgh, K. J. and Wilson, C.: Tide-surge interaction and its role in the distribution of surge residuals in the North Sea, J. Geophys. Res., 112, 2006JC004033, https://doi.org/10.1029/2006JC004033, 2007.
Hou, Y., Yang, J., Russoniello, C. J., Zheng, T., Wu, M., and Yu, X.: Impacts of Coastal Shrimp Ponds on Saltwater Intrusion and Submarine Groundwater Discharge, Water Resour. Res., 58, e2021WR031866, https://doi.org/10.1029/2021WR031866, 2022.
Hugo, G.: Future demographic change and its interactions with migration and climate change, Global Environ. Chang., 21, S21–S33, https://doi.org/10.1016/j.gloenvcha.2011.09.008, 2011.
Huizer, S., Oude Essink, G. H. P., and Bierkens, M. F. P.: Fresh groundwater resources in a large sand replenishment, Hydrol. Earth Syst. Sci., 20, 3149–3166, https://doi.org/10.5194/hess-20-3149-2016, 2016.
Hurrell, J. W.: Decadal Trends in the North Atlantic Oscillation: Regional Temperatures and Precipitation, Science, 269, 676–679, https://doi.org/10.1126/science.269.5224.676, 1995.
Idier, D., Paris, F., Cozannet, G. L., Boulahya, F., and Dumas, F.: Sea-level rise impacts on the tides of the European Shelf, Cont. Shelf Res., 137, 56–71, https://doi.org/10.1016/j.csr.2017.01.007, 2017.
Idier, D., Bertin, X., Thompson, P., and Pickering, M. D.: Interactions Between Mean Sea Level, Tide, Surge, Waves and Flooding: Mechanisms and Contributions to Sea Level Variations at the Coast, Surv. Geophys., 40, 1603–1630, https://doi.org/10.1007/s10712-019-09549-5, 2019.
InCom WG: Saltwater intrusion mitigation in inland waterways, PIANC Report, 198, PIANC, Brussels, 133 pp., ISBN 978-2-87223-014-3, 2021.
Irazoqui Apecechea, M., Melet, A., and Armaroli, C.: Towards a pan-European coastal flood awareness system: Skill of extreme sea-level forecasts from the Copernicus Marine Service, Front. Mar. Sci., 9, 1091844, https://doi.org/10.3389/fmars.2022.1091844, 2023.
Jackson, L. and Devadason, C. A.: Climate Change, Flooding and Mental Health, The Rockefeller Foundation, New York, USA, 2019.
Jiménez, J. A., Osorio, A., Marino-Tapia, I., Davidson, M., Medina, R., Kroon, A., Archetti, R., Ciavola, P., and Aarnikhof, S. G. J.: Beach recreation planning using video-derived coastal state indicators, Coast. Eng., 54, 507–521, https://doi.org/10.1016/j.coastaleng.2007.01.012, 2007.
Ketabchi, H. and Jahangir, M. S.: Influence of aquifer heterogeneity on sea level rise-induced seawater intrusion: A probabilistic approach, J. Contam. Hydrol., 236, 103753, https://doi.org/10.1016/j.jconhyd.2020.103753, 2021.
Ketabchi, H., Mahmoodzadeh, D., Ataie-Ashtiani, B., and Simmons, C. T.: Sea-level rise impacts on seawater intrusion in coastal aquifers: Review and integration, J. Hydrol., 535, 235–255, https://doi.org/10.1016/j.jhydrol.2016.01.083, 2016.
Kew, S. F., Selten, F. M., Lenderink, G., and Hazeleger, W.: The simultaneous occurrence of surge and discharge extremes for the Rhine delta, Nat. Hazards Earth Syst. Sci., 13, 2017–2029, https://doi.org/10.5194/nhess-13-2017-2013, 2013.
Khanal, S., Ridder, N., de Vries, H., Terink, W., and van den Hurk, B.: Storm surge and extreme river discharge: a compound event analysis using ensemble impact modelling, Hydrol. Earth Syst. Sci. Discuss. [preprint], https://doi.org/10.5194/hess-2018-103, 2018.
Komar, P. D.: Beach Processes and Sedimentation, 2nd edn., Prentice Hall, Upper Saddle River (New Jersey), 544 pp., ISBN 9780137549382, 1998.
Kothuis, B. and Kok, M. (Eds.): Integral design of multifunctional flood defenses: multidisciplinary approaches & examples, Delft University Publishers, Delft, the Netherlands, 2017.
Kroon, A., Larson, M., Möller, I., Yokoki, H., Rozynski, G., Cox, J., and Larroude, P.: Statistical analysis of coastal morphological data sets over seasonal to decadal time scales, Coast. Eng., 55, 581–600, https://doi.org/10.1016/j.coastaleng.2007.11.006, 2008.
Kulp, S. A. and Strauss, B. H.: CoastalDEM: A global coastal digital elevation model improved from SRTM using a neural network, Remote Sens. Environ., 206, 231–239, https://doi.org/10.1016/j.rse.2017.12.026, 2018.
Lansu, E. M., Reijers, V. C., Höfer, S., Luijendijk, A., Rietkerk, M., Wassen, M. J., Lammerts, E. J., and Van Der Heide, T.: A global analysis of how human infrastructure squeezes sandy coasts, Nat. Commun., 15, 432, https://doi.org/10.1038/s41467-023-44659-0, 2024.
Larson, M. and Kraus, N. C.: Temporal and spatial scales of beach profile change, Duck, North Carolina, Mar. Geol., 117, 75–94, https://doi.org/10.1016/0025-3227(94)90007-8, 1994.
Leaman, C. K., Harley, M. D., Splinter, K. D., Thran, M. C., Kinsela, M. A., and Turner, I. L.: A storm hazard matrix combining coastal flooding and beach erosion, Coast. Eng., 170, 104001, https://doi.org/10.1016/j.coastaleng.2021.104001, 2021.
Lee, J. J., Phillips, D. L., and Benson, V. W.: Soil erosion and climate change: Assessing potential impacts and adaptation practices, J. Soil Water Conserv., 54, 529–536, 1999.
Le Gal, M., Fernández-Montblanc, T., Montes Perez, J., Souto Ceccon, P. E., Duo, E., Gastal, V., Delbour, S., and Ciavola, P.: A flood map catalogue for integration into aEuropean flood awareness system (ECFAS), EGU General Assembly 2022, Vienna, Austria, 23–27 May 2022, EGU22-9832, https://doi.org/10.5194/egusphere-egu22-9832, 2022.
Lentz, E. E., Thieler, E. R., Plant, N. G., Stippa, S. R., Horton, R. M., and Gesch, D. B.: Evaluation of dynamic coastal response to sea-level rise modifies inundation likelihood, Nat. Clim. Change, 6, 696–700, https://doi.org/10.1038/nclimate2957, 2016.
Lerczak, J. A., Geyer, W. R., and Chant, R. J.: Mechanisms Driving the Time-Dependent Salt Flux in a Partially Stratified Estuary, J. Phys. Oceanogr., 36, 2296–2311, https://doi.org/10.1175/JPO2959.1, 2006.
Lionello, P., Barriopedro, D., Ferrarin, C., Nicholls, R. J., Orlić, M., Raicich, F., Reale, M., Umgiesser, G., Vousdoukas, M., and Zanchettin, D.: Extreme floods of Venice: characteristics, dynamics, past and future evolution (review article), Nat. Hazards Earth Syst. Sci., 21, 2705–2731, https://doi.org/10.5194/nhess-21-2705-2021, 2021.
Liu, Q., Trinder, J., and Turner, I. L.: Automatic super-resolution shoreline change monitoring using Landsat archival data: a case study at Narrabeen–Collaroy Beach, Australia, J. Appl. Remote Sens., 11, 016036, https://doi.org/10.1117/1.JRS.11.016036, 2017.
Loáiciga, H. A.: Long-term climatic change and sustainable ground water resources management, Environ. Res. Lett., 4, 035004, https://doi.org/10.1088/1748-9326/4/3/035004, 2009.
López-Olmedilla, L., Almeida, L. P., de Figueiredo, S. A., Fontán-Bouzas, Á., Silva, P. A., and Alcántara-Carrió, J.: Effect of alongshore sediment supply gradients on projected shoreline position under sea-level rise (northwestern Portuguese coast), Estuar. Coast. Shelf S., 271, 107876, https://doi.org/10.1016/j.ecss.2022.107876, 2022.
Loureiro, C., Ferreira, Ó., and Cooper, J. A. G.: Geologically constrained morphological variability and boundary effects on embayed beaches, Mar. Geol., 329–331, 1–15, https://doi.org/10.1016/j.margeo.2012.09.010, 2012.
Luijendijk, A., Hagenaars, G., Ranasinghe, R., Baart, F., Donchyts, G., and Aarninkhof, S.: The State of the World's Beaches, Sci. Rep., 8, 6641, https://doi.org/10.1038/s41598-018-24630-6, 2018.
Luque, P., Gómez-Pujol, L., Marcos, M., and Orfila, A.: Coastal Flooding in the Balearic Islands During the Twenty-First Century Caused by Sea-Level Rise and Extreme Events, Front. Mar. Sci., 8, 676452, https://doi.org/10.3389/fmars.2021.676452, 2021.
Maas, E. V. and Hoffman, G. J.: Crop Salt Tolerance – Current Assessment, J. Irrig. Drain. Div., 103, 115–134, https://doi.org/10.1061/JRCEA4.0001137, 1977.
Mabrouk, M., Jonoski, A., H. P. Oude Essink, G., and Uhlenbrook, S.: Impacts of Sea Level Rise and Groundwater Extraction Scenarios on Fresh Groundwater Resources in the Nile Delta Governorates, Egypt, Water, 10, 1690, https://doi.org/10.3390/w10111690, 2018.
Malenovský, Z., Rott, H., Cihlar, J., Schaepman, M. E., García-Santos, G., Fernandes, R., and Berger, M.: Sentinels for science: Potential of Sentinel-1, -2, and -3 missions for scientific observations of ocean, cryosphere, and land, Remote Sens. Environ., 120, 91–101, https://doi.org/10.1016/j.rse.2011.09.026, 2012.
Mantz, P. and Wakeling, H.: Forecasting flood levels for joint events of rainfall and tidal surge flooding using extreme value statistics, P. I. Civil Eng., 67, 31–50, https://doi.org/10.1680/iicep.1979.2315, 1979.
Marcos, M., Calafat, F. M., Berihuete, Á., and Dangendorf, S.: Long-term variations in global sea level extremes, J. Geophys. Res.-Oceans, 120, 8115–8134, https://doi.org/10.1002/2015JC011173, 2015.
Marcos, M., Wöppelmann, G., Matthews, A., Ponte, R. M., Birol, F., Ardhuin, F., Coco, G., Santamaría-Gómez, A., Ballu, V., Testut, L., Chambers, D., and Stopa, J. E.: Coastal Sea Level and Related Fields from Existing Observing Systems, Surv. Geophys., 40, 1293–1317, https://doi.org/10.1007/s10712-019-09513-3, 2019.
Maselli, V. and Trincardi, F.: Man made deltas, Sci. Rep., 3, 1926, https://doi.org/10.1038/srep01926, 2013.
Masselink, G., Brooks, S., Poate, T., Stokes, C., and Scott, T.: Coastal dune dynamics in embayed settings with sea-level rise – Examples from the exposed and macrotidal north coast of SW England, Mar. Geol., 450, 106853, https://doi.org/10.1016/j.margeo.2022.106853, 2022.
Masterson, J. P. and Garabedian, S. P.: Effects of Sea-Level Rise on Ground Water Flow in a Coastal Aquifer System, Ground Water, 45, 209–217, https://doi.org/10.1111/j.1745-6584.2006.00279.x, 2007.
Mastrocicco, M. and Colombani, N.: The Issue of Groundwater Salinization in Coastal Areas of the Mediterranean Region: A Review, Water, 13, 90, https://doi.org/10.3390/w13010090, 2021.
Mazas, F. and Hamm, L.: An event-based approach for extreme joint probabilities of waves and sea levels, Coast. Eng., 122, 44–59, https://doi.org/10.1016/j.coastaleng.2017.02.003, 2017.
Mazi, K., Koussis, A. D., and Destouni, G.: Intensively exploited Mediterranean aquifers: resilience to seawater intrusion and proximity to critical thresholds, Hydrol. Earth Syst. Sci., 18, 1663–1677, https://doi.org/10.5194/hess-18-1663-2014, 2014.
McCall, R. T., Van Thiel De Vries, J. S. M., Plant, N. G., Van Dongeren, A. R., Roelvink, J. A., Thompson, D. M., and Reniers, A. J. H. M.: Two-dimensional time dependent hurricane overwash and erosion modeling at Santa Rosa Island, Coast. Eng., 57, 668–683, https://doi.org/10.1016/j.coastaleng.2010.02.006, 2010.
McGranahan, G., Balk, D., and Anderson, B.: The rising tide: assessing the risks of climate change and human settlements in low elevation coastal zones, Environ. Urban., 19, 17–37, https://doi.org/10.1177/0956247807076960, 2007.
McMichael, C., Dasgupta, S., Ayeb-Karlsson, S., and Kelman, I.: A review of estimating population exposure to sea-level rise and the relevance for migration, Environ. Res. Lett., 15, 123005, https://doi.org/10.1088/1748-9326/abb398, 2020.
Mel, R. A., Carniello, L., and D'Alpaos, L.: How long the Mo.S.E. barriers will be effective in protecting all urban settlements within the Venice Lagoon? The wind setup constraint, Coast. Eng., 168, 103923, https://doi.org/10.1016/j.coastaleng.2021.103923, 2021.
Melet, A., Almar, R., Hemer, M., Le Cozannet, G., Meyssignac, B., and Ruggiero, P.: Contribution of Wave Setup to Projected Coastal Sea Level Changes, J. Geophys. Res.-Oceans, 125, e2020JC016078, https://doi.org/10.1029/2020JC016078, 2020.
Melet, A., Buontempo, C., Mattiuzzi, M., Salamon, P., Bahurel, P., Breyiannis, G., Burgess, S., Crosnier, L., Le Traon, P.-Y., Mentaschi, L., Nicolas, J., Solari, L., Vamborg, F., and Voukouvalas, E.: European Copernicus Services to Inform on Sea-Level Rise Adaptation: Current Status and Perspectives, Front. Mar. Sci., 8, 703425, https://doi.org/10.3389/fmars.2021.703425, 2021.
Melet, A., van de Wal, R., Amores, A., Arns, A., Chaigneau, A. A., Dinu, I., Haigh, I. D., Hermans, T. H. J., Lionello, P., Marcos, M., Meier, H. E. M., Meyssignac, B., Palmer, M. D., Reese, R., Simpson, M. J. R., and Slangen, A. B. A.: Sea Level Rise in Europe: Observations and projections, in: Sea Level Rise in Europe: 1st Assessment Report of the Knowledge Hub on Sea Level Rise (SLRE1), edited by: van den Hurk, B., Pinardi, N., Kiefer, T., Larkin, K., Manderscheid, P., and Richter, K., Copernicus Publications, State Planet, 3-slre1, 4, https://doi.org/10.5194/sp-3-slre1-4-2024, 2024.
Meli, M. and Romagnoli, C.: Evidence and Implications of Hydrological and Climatic Change in the Reno and Lamone River Basins and Related Coastal Areas (Emilia-Romagna, Northern Italy) over the Last Century, Water, 14, 2650, https://doi.org/10.3390/w14172650, 2022.
Meli, M., Camargo, C. M. L., Olivieri, M., Slangen, A. B. A., and Romagnoli, C.: Sea-level trend variability in the Mediterranean during the 1993–2019 period, Front. Mar. Sci., 10, 1150488, https://doi.org/10.3389/fmars.2023.1150488, 2023.
Menéndez, M. and Woodworth, P. L.: Changes in extreme high water levels based on a quasi-global tide-gauge data set, J. Geophys. Res., 115, C10011, https://doi.org/10.1029/2009JC005997, 2010.
Menna, M., Gačić, M., Martellucci, R., Notarstefano, G., Fedele, G., Mauri, E., Gerin, R., and Poulain, P.-M.: Climatic, Decadal, and Interannual Variability in the Upper Layer of the Mediterranean Sea Using Remotely Sensed and In-Situ Data, Remote Sens., 14, 1322, https://doi.org/10.3390/rs14061322, 2022.
Mentaschi, L., Vousdoukas, M. I., Voukouvalas, E., Dosio, A., and Feyen, L.: Global changes of extreme coastal wave energy fluxes triggered by intensified teleconnection patterns, Geophys. Res. Lett., 44, 2416–2426, https://doi.org/10.1002/2016GL072488, 2017.
Mentaschi, L., Vousdoukas, M. I., Pekel, J.-F., Voukouvalas, E., and Feyen, L.: Global long-term observations of coastal erosion and accretion, Sci. Rep., 8, 12876, https://doi.org/10.1038/s41598-018-30904-w, 2018.
Michael, H. A., Russoniello, C. J., and Byron, L. A.: Global assessment of vulnerability to sea-level rise in topography-limited and recharge-limited coastal groundwater systems, Water Resour. Res., 49, 2228–2240, https://doi.org/10.1002/wrcr.20213, 2013.
Milliman, J. D.: Blessed dams or damned dams?, Nature, 386, 325–327, https://doi.org/10.1038/386325a0, 1997.
Minderhoud, P. S. J., Erkens, G., Pham, V. H., Bui, V. T., Erban, L., Kooi, H., and Stouthamer, E.: Impacts of 25 years of groundwater extraction on subsidence in the Mekong delta, Vietnam, Environ. Res. Lett., 12, 064006, https://doi.org/10.1088/1748-9326/aa7146, 2017.
Moftakhari, H. R., Salvadori, G., AghaKouchak, A., Sanders, B. F., and Matthew, R. A.: Compounding effects of sea level rise and fluvial flooding, P. Natl. Acad. Sci. USA, 114, 9785–9790, https://doi.org/10.1073/pnas.1620325114, 2017.
Moftakhari, H. R., AghaKouchak, A., Sanders, B. F., Allaire, M., and Matthew, R. A.: What Is Nuisance Flooding? Defining and Monitoring an Emerging Challenge, Water Resour. Res., 54, 4218–4227, https://doi.org/10.1029/2018WR022828, 2018.
Monioudi, I. N., Velegrakis, A. F., Chatzipavlis, A. E., Rigos, A., Karambas, T., Vousdoukas, M. I., Hasiotis, T., Koukourouvli, N., Peduzzi, P., Manoutsoglou, E., Poulos, S. E., and Collins, M. B.: Assessment of island beach erosion due to sea level rise: the case of the Aegean archipelago (Eastern Mediterranean), Nat. Hazards Earth Syst. Sci., 17, 449–466, https://doi.org/10.5194/nhess-17-449-2017, 2017.
Mooyaart, L. F. and Jonkman, S. N.: Overview and Design Considerations of Storm Surge Barriers, J. Waterw. Port Coast., 143, 06017001, https://doi.org/10.1061/(ASCE)WW.1943-5460.0000383, 2017.
Morim, J., Hemer, M., Wang, X. L., Cartwright, N., Trenham, C., Semedo, A., Young, I., Bricheno, L., Camus, P., Casas-Prat, M., Erikson, L., Mentaschi, L., Mori, N., Shimura, T., Timmermans, B., Aarnes, O., Breivik, O., Behrens, A., Dobrynin, M., Menendez, M., Staneva, J., Wehner, M., Wolf, J., Kamranzad, B., Webb, A., Stopa, J., and Andutta, F.: Robustness and uncertainties in global multivariate wind-wave climate projections, Nat. Clim. Change, 9, 711–718, https://doi.org/10.1038/s41558-019-0542-5, 2019.
Muis, S., Apecechea, M. I., Dullaart, J., de Lima Rego, J., Madsen, K. S., Su, J., Yan, K., and Verlaan, M.: A High-Resolution Global Dataset of Extreme Sea Levels, Tides, and Storm Surges, Including Future Projections, Front. Mar. Sci., 7, 263, https://doi.org/10.3389/fmars.2020.00263, 2020.
Murray, A. B., Lazarus, E., Ashton, A., Baas, A., Coco, G., Coulthard, T., Fonstad, M., Haff, P., McNamara, D., Paola, C., Pelletier, J., and Reinhardt, L.: Geomorphology, complexity, and the emerging science of the Earth's surface, Geomorphology, 103, 496–505, https://doi.org/10.1016/j.geomorph.2008.08.013, 2009.
Navoy, A. S.: Aquifer-estuary interaction and vulnerability of groundwater supplies to sea level rise-driven saltwater intrusion, Pennsylvania State University, United States of America, 225 pp., 1991.
Neumann, B., Vafeidis, A. T., Zimmermann, J., and Nicholls, R. J.: Future Coastal Population Growth and Exposure to Sea-Level Rise and Coastal Flooding – A Global Assessment, PLOS ONE, 10, e0118571, https://doi.org/10.1371/journal.pone.0118571, 2015.
Nicholls, R., Wong, P., Burkett, V., Codignotto, J., Hay, J., McLean, R., and Woodroffe, S. R. C.: Coastal systems and low-lying areas, in: Climate Change 2007: Impacts, Adaptation and Vulnerability. Contribution of Working Group II to the Fourth Assessment Report of the Intergovernmental Panel on Climate Change, edited by: Parry, M. L., Canziani, O. F., Palutikof, J. P., van der Linden, P. J., and Hanson, C. E., Cambridge University Press, Cambridge, UK, 315–356, 2007.
Nicholls, R. J. and Cazenave, A.: Sea-Level Rise and Its Impact on Coastal Zones, Science, 328, 1517–1520, https://doi.org/10.1126/science.1185782, 2010.
Noble, R. M.: Coastal structures’ effects on shorelines, Coast. Eng., 1978, 2068–2085, https://doi.org/10.1061/9780872621909.127, 1978.
Oppenheimer, M., Glavovic, B., Hinkel, J., van de Wal, R. S. W., Magnan, A., Abd-Elgawad, A., Cai, R., Cifuentes-Jara, M., DeConto, R. M., Ghosh, T., Hay, J., Isla, F. I., Marzeion, B., Meyssignac, B., and Sebesvari, Z.: Sea Level Rise and Implications for Low Lying Islands, Coasts and Communities, in: IPCC Special Report on the Ocean and Cryosphere in a Changing Climate, edited by: Pörtner, H.-O., Roberts, D. C., Masson-Delmotte, V., Zhai, P., Tignor, M., Poloczanska, E., Mintenbeck, K., Alegría, A., Nicolai, M., Okem, A., Petzold, J., Rama, B., and Weyer, N. M., Cambridge University Press, Cambridge, UK, https://doi.org/10.1017/9781009157964.006, 2019.
Oude Essink, G. H. P.: Impact of sea level rise on groundwater flow regimes: a sensitivity analysis for the Netherlands, Delft University Press, Delft, 411 pp., ISBN 90-407-1330-8, 1996.
Oude Essink, G. H. P.: Salt Water Intrusion in a Three-dimensional Groundwater System in The Netherlands: A Numerical Study, Transport Porous Med., 43, 137–158, https://doi.org/10.1023/A:1010625913251, 2001.
Oude Essink, G. H. P., Van Baaren, E. S., and De Louw, P. G. B.: Effects of climate change on coastal groundwater systems: A modeling study in the Netherlands, Water Resour. Res., 46, 2009WR008719, https://doi.org/10.1029/2009WR008719, 2010.
Paprotny, D., Morales-Nápoles, O., and Jonkman, S. N.: HANZE: a pan-European database of exposure to natural hazards and damaging historical floods since 1870, Earth Syst. Sci. Data, 10, 565–581, https://doi.org/10.5194/essd-10-565-2018, 2018.
Paprotny, D., Morales-Nápoles, O., Vousdoukas, M. I., Jonkman, S. N., and Nikulin, G.: Accuracy of pan-European coastal flood mapping, J. Flood Risk Manage., 12, e12459, https://doi.org/10.1111/jfr3.12459, 2019.
Paprotny, D., Vousdoukas, M. I., Morales-Nápoles, O., Jonkman, S. N., and Feyen, L.: Pan-European hydrodynamic models and their ability to identify compound floods, Nat. Hazards, 101, 933–957, https://doi.org/10.1007/s11069-020-03902-3, 2020.
Paprotny, D., Terefenko, P., Giza, A., Czapliński, P., and Vousdoukas, M. I.: Future losses of ecosystem services due to coastal erosion in Europe, Sci. Total Environ., 760, 144310, https://doi.org/10.1016/j.scitotenv.2020.144310, 2021.
Passeri, D. L., Hagen, S. C., Bilskie, M. V., and Medeiros, S. C.: On the significance of incorporating shoreline changes for evaluating coastal hydrodynamics under sea level rise scenarios, Nat. Hazards, 75, 1599–1617, https://doi.org/10.1007/s11069-014-1386-y, 2015a.
Passeri, D. L., Hagen, S. C., Medeiros, S. C., Bilskie, M. V., Alizad, K., and Wang, D.: The dynamic effects of sea level rise on low-gradient coastal landscapes: A review, Earths Future, 3, 159–181, https://doi.org/10.1002/2015EF000298, 2015b.
Passeri, D. L., Hagen, S. C., Plant, N. G., Bilskie, M. V., Medeiros, S. C., and Alizad, K.: Tidal hydrodynamics under future sea level rise and coastal morphology in the Northern Gulf of Mexico, Earths Future, 4, 159–176, https://doi.org/10.1002/2015EF000332, 2016.
Passeri, D. L., Bilskie, M. V., Plant, N. G., Long, J. W., and Hagen, S. C.: Dynamic modeling of barrier island response to hurricane storm surge under future sea level rise, Climatic Change, 149, 413–425, https://doi.org/10.1007/s10584-018-2245-8, 2018.
Pereira, H., Sousa, M. C., Vieira, L. R., Morgado, F., and Dias, J. M.: Modelling Salt Intrusion and Estuarine Plumes under Climate Change Scenarios in Two Transitional Ecosystems from the NW Atlantic Coast, Journal of Marine Science and Engineering, 10, 262, https://doi.org/10.3390/jmse10020262, 2022.
Petroliagkis, T. I., Bidlot, J., Voukouvalas, E., and Disperati, J.: Joint probabilities of storm surge, significant wave height and river discharge components of coastal flooding events: utilising statistical dependence methodologies & techniques, Publications Office of the European Union, LU, https://doi.org/10.2788/951583, 2016.
Pickering, M. D., Wells, N. C., Horsburgh, K. J., and Green, J. A. M.: The impact of future sea-level rise on the European Shelf tides, Cont. Shelf Res., 35, 1–15, https://doi.org/10.1016/j.csr.2011.11.011, 2012.
Pietro, L. S., O'Neal, M. A., and Puleo, J. A.: Developing Terrestrial-LIDAR-Based Digital Elevation Models for Monitoring Beach Nourishment Performance, J. Coastal Res., 246, 1555–1564, https://doi.org/10.2112/07-0904.1, 2008.
Pollard, J., Spencer, T., and Brooks, S.: The interactive relationship between coastal erosion and flood risk, Progress in Physical Geography: Earth and Environment, 43, 574–585, https://doi.org/10.1177/0309133318794498, 2019.
Pörtner, H.-O., Roberts, C. D., Poloczanska, E., Mintenbeck, K., Tignor, M., Alegria, A., Craig, M., Langsdorf, S., Löschke, S., Möller, V., and Okem, A. (Eds.): IPCC Summary for Policymakers, in: Climate Change 2022: Impacts, Adaptation and Vulnerability. Contribution of Working Group II to the Sixth Assessment Report of the Intergovernmental Panel on Climate Change, Cambridge University Press, Cambridge, UK and New York, NY, USA, 3–33, https://doi.org//10.1017/9781009157964.001, 2022.
Post, V. E. A., Oude Essink, G., Szymkiewicz, A., Bakker, M., Houben, G., Custodio, E., and Voss, C.: Celebrating 50 years of SWIMs (Salt Water Intrusion Meetings), Hydrogeol. J., 26, 1767–1770, https://doi.org/10.1007/s10040-018-1800-8, 2018.
Pugh, D. and Woodworth, P.: Sea-Level Science: Understanding Tides, Surges, Tsunamis and Mean Sea-Level Changes, 1st edn., Cambridge University Press, https://doi.org/10.1017/CBO9781139235778, 2014.
Qadir, M., Quillérou, E., Nangia, V., Murtaza, G., Singh, M., Thomas, R. J., Drechsel, P., and Noble, A. D.: Economics of salt-induced land degradation and restoration, Nat. Resour. Forum, 38, 282–295, https://doi.org/10.1111/1477-8947.12054, 2014.
Qi, H., Cai, F., Lei, G., Cao, H., and Shi, F.: The response of three main beach types to tropical storms in South China, Mar. Geol., 275, 244–254, https://doi.org/10.1016/j.margeo.2010.06.005, 2010.
Rasmussen, P., Sonnenborg, T. O., Goncear, G., and Hinsby, K.: Assessing impacts of climate change, sea level rise, and drainage canals on saltwater intrusion to coastal aquifer, Hydrol. Earth Syst. Sci., 17, 421–443, https://doi.org/10.5194/hess-17-421-2013, 2013.
Reimann, L., Vafeidis, A. T., Brown, S., Hinkel, J., and Tol, R. S.: Mediterranean UNESCO World Heritage at risk from coastal flooding and erosion due to sea-level rise, Nat. Commun., 9, 4161, https://doi.org/10.1038/s41467-018-06645-9, 2018.
Rodrigues, M., Fortunato, A. B., and Freire, P.: Saltwater Intrusion in the Upper Tagus Estuary during Droughts, Geosciences, 9, 400, https://doi.org/10.3390/geosciences9090400, 2019.
Roest, B., de Vries, S., de Schipper, M., and Aarninkhof, S.: Observed Changes of a Mega Feeder Nourishment in a Coastal Cell: Five Years of Sand Engine Morphodynamics, Journal of Marine Science and Engineering, 9, 37, https://doi.org/10.3390/jmse9010037, 2021.
Romagnoli, C., Bosman, A., Casalbore, D., Anzidei, M., Doumaz, F., Bonaventura, F., Meli, M., and Verdirame, C.: Coastal Erosion and Flooding Threaten Low-Lying Coastal Tracts at Lipari (Aeolian Islands, Italy), Remote Sens., 14, 2960, https://doi.org/10.3390/rs14132960, 2022.
Rueda, A., Camus, P., Tomás, A., Vitousek, S., and Méndez, F. J.: A multivariate extreme wave and storm surge climate emulator based on weather patterns, Ocean Model., 104, 242–251, https://doi.org/10.1016/j.ocemod.2016.06.008, 2016.
Ruocco, A. C., Nicholls, R. J., Haigh, I. D., and Wadey, M. P.: Reconstructing coastal flood occurrence combining sea level and media sources: a case study of the Solent, UK since 1935, Nat. Hazards, 59, 1773–1796, https://doi.org/10.1007/s11069-011-9868-7, 2011.
Russ, J., Zaveri, E., Damania, R., Desbureaux, S., Escurra, J., and Rodella, A.-S.: Salt of the Earth: Quantifying the Impact of Water Salinity on Global Agricultural Productivity, World Bank, Washington, DC, https://doi.org/10.1596/1813-9450-9144, 2020.
Sabour, S., Brown, S., Nicholls, R. J., Haigh, I. D., and Luijendijk, A. P.: Multi-decadal shoreline change in coastal natural world heritage sites – a global assessment, Environ. Res. Lett., 15, 104047, https://doi.org/10.1088/1748-9326/ab968f, 2020.
Sallenger, A. H. Jr.: Storm Impact Scale for Barrier Islands, J. Coastal Res., 16, 890–895, 2000.
Samuels, P. G. and Burt, N.: A new joint probability appraisal of flood risk, P. I. Civil Eng.-Water, 154, 109–115, https://doi.org/10.1680/wame.2002.154.2.109, 2002.
Santos, V. M., Casas-Prat, M., Poschlod, B., Ragno, E., van den Hurk, B., Hao, Z., Kalmár, T., Zhu, L., and Najafi, H.: Statistical modelling and climate variability of compound surge and precipitation events in a managed water system: a case study in the Netherlands, Hydrol. Earth Syst. Sci., 25, 3595–3615, https://doi.org/10.5194/hess-25-3595-2021, 2021.
Sanuy, M. and Jiménez, J. A.: Probabilistic characterisation of coastal storm-induced risks using Bayesian networks, Nat. Hazards Earth Syst. Sci., 21, 219–238, https://doi.org/10.5194/nhess-21-219-2021, 2021.
Sayers, P. B., Hall, J. W., and Meadowcroft, I. C.: Towards risk-based flood hazard management in the UK, P. I. Civil Eng.-Civ. Eng., 150, 36–42, https://doi.org/10.1680/cien.2002.150.5.36, 2002.
Sayers, P. B., Horritt, M. S., Penning-Rowsell, E., and McKenzie, A.: Climate Change Risk Assessment 2017: Projections of future flood risk in the UK. Research undertaken by Sayers and Partners on behalf of the Committee on Climate Change, Committee on Climate Change, London, https://www.theccc.org.uk/wp-content/uploads/2015/10/CCRA-Future-Flooding-Main-Report-Final-06Oct2015.pdf.pdf (last access: 14 July 2024), 2015.
Schmork, S. and Mercado, A.: Upconing of Fresh Water-Sea Water Interface Below Pumping Wells, Field Study, Water Resour. Res., 5, 1290–1311, https://doi.org/10.1029/WR005i006p01290, 1969.
Scussolini, P., Aerts, J. C. J. H., Jongman, B., Bouwer, L. M., Winsemius, H. C., de Moel, H., and Ward, P. J.: FLOPROS: an evolving global database of flood protection standards, Nat. Hazards Earth Syst. Sci., 16, 1049–1061, https://doi.org/10.5194/nhess-16-1049-2016, 2016.
Sherif, M. M. and Singh, V. P.: Effect of climate change on sea water intrusion in coastal aquifers, Hydrol. Process., 13, 1277–1287, https://doi.org/10.1002/(SICI)1099-1085(19990615)13:8<1277::AID-HYP765>3.0.CO;2-W, 1999.
Short, A. D. (Ed.): Handbook of beach and shoreface morphodynamics, John Wiley, New York, 379 pp., ISBN 0-471-965707, 1999.
Short, A. D. and Trembanis, A. C.: Decadal Scale Patterns in Beach Oscillation and Rotation Narrabeen Beach, Australia – Time Series, PCA and Wavelet Analysis, J. Coastal Res., 202, 523–532, https://doi.org/10.2112/1551-5036(2004)020[0523:DSPIBO]2.0.CO;2, 2004.
Siemon, B., Costabel, S., Voß, W., Meyer, U., Deus, N., Elbracht, J., Günther, T., and Wiederhold, H.: Airborne and ground geophysical mapping of coastal clays in Eastern Friesland, Germany, Geophysics, 80, WB21–WB34, https://doi.org/10.1190/geo2014-0102.1, 2015.
Sierra, J. P. and Casas-Prat, M.: Analysis of potential impacts on coastal areas due to changes in wave conditions, Climatic Change, 124, 861–876, https://doi.org/10.1007/s10584-014-1120-5, 2014.
Silva, R., Martínez, M. L., Van Tussenbroek, B. I., Guzmán-Rodríguez, L. O., Mendoza, E., and López-Portillo, J.: A Framework to Manage Coastal Squeeze, Sustainability, 12, 10610, https://doi.org/10.3390/su122410610, 2020.
Small, C. and Nicholls, R. J.: A global analysis of human settlement in coastal zones, J. Coastal Res., 19, 584–599, 2003.
Sprenger, C., Hartog, N., Hernández, M., Vilanova, E., Grützmacher, G., Scheibler, F., and Hannappel, S.: Inventory of managed aquifer recharge sites in Europe: historical development, current situation and perspectives, Hydrogeol. J., 25, 1909–1922, https://doi.org/10.1007/s10040-017-1554-8, 2017.
Stanghellini, G., Bidini, C., Romagnoli, C., Archetti, R., Ponti, M., Turicchia, E., Del Bianco, F., Mercorella, A., Polonia, A., Giorgetti, G., Gallerani, A., and Gasperini, L.: Repeated (4D) Marine Geophysical Surveys as a Tool for Studying the Coastal Environment and Ground-Truthing Remote-Sensing Observations and Modeling, Remote Sens., 14, 5901, https://doi.org/10.3390/rs14225901, 2022.
Stevens, A. J., Clarke, D., and Nicholls, R. J.: Trends in reported flooding in the UK: 1884–2013, Hydrolog. Sci. J., 61, 50–63, https://doi.org/10.1080/02626667.2014.950581, 2016.
Stofberg, S. F., Klimkowska, A., Paulissen, M. P. C. P., Witte, J.-P. M., and van der Zee, S. E. A. T. M.: Effects of salinity on growth of plant species from terrestrializing fens, Aquat. Bot., 121, 83–90, https://doi.org/10.1016/j.aquabot.2014.12.004, 2015.
Stringari, C. E. and Power, H.: Picoastal: A low-cost coastal video monitoring system, SoftwareX, 18, 101073, https://doi.org/10.1016/j.softx.2022.101073, 2022.
Stripling, S., Panzeri, M., Blanco, B., Rossington, K., Sayers, P., and Borthwick, A.: Regional-scale probabilistic shoreline evolution modelling for flood-risk assessment, Coast. Eng., 121, 129–144, https://doi.org/10.1016/j.coastaleng.2016.12.002, 2017.
Svensson, C. and Jones, D. A.: Dependence between extreme sea surge, river flow and precipitation in eastern Britain, Int. J. Climatol., 22, 1149–1168, https://doi.org/10.1002/joc.794, 2002.
Svensson, C. and Jones, D. A.: Dependence between sea surge, river flow and precipitation in south and west Britain, Hydrol. Earth Syst. Sci., 8, 973–992, https://doi.org/10.5194/hess-8-973-2004, 2004.
Taylor, R. G., Scanlon, B., Döll, P., Rodell, M., van Beek, R., Wada, Y., Longuevergne, L., Leblanc, M., Famiglietti, J. S., Edmunds, M., Konikow, L., Green, T. R., Chen, J., Taniguchi, M., Bierkens, M. F. P., MacDonald, A., Fan, Y., Maxwell, R. M., Yechieli, Y., Gurdak, J. J., Allen, D. M., Shamsudduha, M., Hiscock, K., Yeh, P. J.-F., Holman, I., and Treidel, H.: Ground water and climate change, Nat. Clim. Change, 3, 322–329, https://doi.org/10.1038/nclimate1744, 2013.
Thiéblemont, R., Le Cozannet, G., Toimil, A., Meyssignac, B., and Losada, I. J.: Likely and High-End Impacts of Regional Sea-Level Rise on the Shoreline Change of European Sandy Coasts Under a High Greenhouse Gas Emissions Scenario, Water, 11, 2607, https://doi.org/10.3390/w11122607, 2019.
Thompson, G. and Law, F. M.: An assessment of the fluvial tidal flooding problem of the River Ancholme, UK, in: Proceedings from the IUGG Interdisciplinary Symposium on Assessment of Natural Hazards, 15–27 August 1983, Hamburg, Germany, IUGG (International Union of Geodesy and Geophysics), ISBN 978-1-906698-01-0, 1983.
Thorne, C. R., Evans, E. P., and Penning-Rowsell, E. C. (Eds.): Future flooding and coastal erosion risks, Thomas Telford Publishing, https://doi.org/10.1680/ffacer.34495, 2007.
Toimil, A., Losada, I. J., Díaz-Simal, P., Izaguirre, C., and Camus, P.: Multi-sectoral, high-resolution assessment of climate change consequences of coastal flooding, Climatic Change, 145, 431–444, https://doi.org/10.1007/s10584-017-2104-z, 2017.
Toimil, A., Losada, I. J., Nicholls, R. J., Dalrymple, R. A., and Stive, M. J. F.: Addressing the challenges of climate change risks and adaptation in coastal areas: A review, Coast. Eng., 156, 103611, https://doi.org/10.1016/j.coastaleng.2019.103611, 2020.
Toimil, A., Camus, P., Losada, I. J., and Alvarez-Cuesta, M.: Visualising the Uncertainty Cascade in Multi-Ensemble Probabilistic Coastal Erosion Projections, Front. Mar. Sci., 8, 683535, https://doi.org/10.3389/fmars.2021.683535, 2021.
Toimil, A., Losada, I. J., Álvarez-Cuesta, M., and Le Cozannet, G.: Demonstrating the value of beaches for adaptation to future coastal flood risk, Nat. Commun., 14, 3474, https://doi.org/10.1038/s41467-023-39168-z, 2023a.
Toimil, A., Álvarez-Cuesta, M., and Losada, I. J.: Neglecting the effect of long- and short-term erosion can lead to spurious coastal flood risk projections and maladaptation, Coast. Eng., 179, 104248, https://doi.org/10.1016/j.coastaleng.2022.104248, 2023b.
Valle-Levinson, A.: Definition and classification of estuaries, in: Contemporary Issues in Estuarine Physics, edited by: Valle-Levinson, A., Cambridge University Press, 1–11, https://doi.org/10.1017/CBO9780511676567.002, 2010.
Vandenbohede, A., Luyten, K., and Lebbe, L.: Effects of Global Change on Heterogeneous Coastal Aquifers: A Case Study in Belgium, J. Coastal Res., 2, 160–170, https://doi.org/10.2112/05-0447.1, 2008.
Van Den Hurk, B., Van Meijgaard, E., De Valk, P., Van Heeringen, K.-J., and Gooijer, J.: Analysis of a compounding surge and precipitation event in the Netherlands, Environ. Res. Lett., 10, 035001, https://doi.org/10.1088/1748-9326/10/3/035001, 2015.
van Engelen, J., Essink, G. H. P. O., and Bierkens, M. F. P.: Sustainability of fresh groundwater resources in fifteen major deltas around the world, Environ. Res. Lett., 17, 125001, https://doi.org/10.1088/1748-9326/aca16c, 2022.
Van Ormondt, M., Nelson, T. R., Hapke, C. J., and Roelvink, D.: Morphodynamic modelling of the wilderness breach, Fire Island, New York. Part I: Model set-up and validation, Coast. Eng., 157, 103621, https://doi.org/10.1016/j.coastaleng.2019.103621, 2020.
Van Weert, F., Van der Gun, J., and Reckman, J. W. T. M.: Global overview of saline groundwater occurrence and genesis, International Groundwater Resources Assessment Centre, Utrecht, Report no. GP 2009-1, 104 pp., 2009.
Villatoro, M., Silva, R., Méndez, F. J., Zanuttigh, B., Pan, S., Trifonova, E., Losada, I. J., Izaguirre, C., Simmonds, D., Reeve, D. E., Mendoza, E., Martinelli, L., Formentin, S. M., Galiatsatou, P., and Eftimova, P.: An approach to assess flooding and erosion risk for open beaches in a changing climate, Coast. Eng., 87, 50–76, https://doi.org/10.1016/j.coastaleng.2013.11.009, 2014.
Vousdoukas, M. I., Velegrakis, A. F., and Plomaritis, T. A.: Beachrock occurrence, characteristics, formation mechanisms and impacts, Earth-Sci. Rev., 85, 23–46, https://doi.org/10.1016/j.earscirev.2007.07.002, 2007.
Vousdoukas, M. I., Velegrakis, A. F., and Karambas, T. V.: Morphology and sedimentology of a microtidal beach with beachrocks: Vatera, Lesbos, NE Mediterranean, Cont. Shelf Res., 29, 1937–1947, https://doi.org/10.1016/j.csr.2009.04.003, 2009.
Vousdoukas, M. I., Pennucci, G., Holman, R. A., and Conley, D. C.: A semi automatic technique for Rapid Environmental Assessment in the coastal zone using Small Unmanned Aerial Vehicles (SUAV), J. Coastal Res., SI64, 1755–1759, 2011.
Vousdoukas, M. I., Almeida, L. P. M., and Ferreira, Ó.: Beach erosion and recovery during consecutive storms at a steep-sloping, meso-tidal beach, Earth Surf. Proc. Land., 37, 583–593, https://doi.org/10.1002/esp.2264, 2012.
Vousdoukas, M. I., Kirupakaramoorthy, T., Oumeraci, H., de la Torre, M., Wübbold, F., Wagner, B., and Schimmels, S.: The role of combined laser scanning and video techniques in monitoring wave-by-wave swash zone processes, Coast. Eng., 83, 150–165, https://doi.org/10.1016/j.coastaleng.2013.10.013, 2014.
Vousdoukas, M. I., Voukouvalas, E., Annunziato, A., Giardino, A., and Feyen, L.: Projections of extreme storm surge levels along Europe, Clim. Dynam., 47, 3171–3190, https://doi.org/10.1007/s00382-016-3019-5, 2016.
Vousdoukas, M. I., Mentaschi, L., Voukouvalas, E., Verlaan, M., and Feyen, L.: Extreme sea levels on the rise along Europe's coasts, Earths Future, 5, 304–323, https://doi.org/10.1002/2016EF000505, 2017.
Vousdoukas, M. I., Mentaschi, L., Voukouvalas, E., Bianchi, A., Dottori, F., and Feyen, L.: Climatic and socioeconomic controls of future coastal flood risk in Europe, Nat. Clim. Change, 8, 776–780, https://doi.org/10.1038/s41558-018-0260-4, 2018a.
Vousdoukas, M. I., Mentaschi, L., Voukouvalas, E., Verlaan, M., Jevrejeva, S., Jackson, L. P., and Feyen, L.: Global probabilistic projections of extreme sea levels show intensification of coastal flood hazard, Nat. Commun., 9, 2360, https://doi.org/10.1038/s41467-018-04692-w, 2018b.
Vousdoukas, M. I., Ranasinghe, R., Mentaschi, L., Plomaritis, T. A., Athanasiou, P., Luijendijk, A., and Feyen, L.: Sandy coastlines under threat of erosion, Nat. Clim. Change, 10, 260–263, https://doi.org/10.1038/s41558-020-0697-0, 2020.
Vousdoukas, M. I., Clarke, J., Ranasinghe, R., Reimann, L., Khalaf, N., Duong, T. M., Ouweneel, B., Sabour, S., Iles, C. E., Trisos, C. H., Feyen, L., Mentaschi, L., and Simpson, N. P.: African heritage sites threatened as sea-level rise accelerates, Nat. Clim. Change, 12, 256–262, https://doi.org/10.1038/s41558-022-01280-1, 2022.
Wada, Y., Van Beek, L. P. H., Van Kempen, C. M., Reckman, J. W. T. M., Vasak, S., and Bierkens, M. F. P.: Global depletion of groundwater resources, Geophys. Res. Lett., 37, L20402, https://doi.org/10.1029/2010GL044571, 2010.
Wada, Y., Wisser, D., and Bierkens, M. F. P.: Global modeling of withdrawal, allocation and consumptive use of surface water and groundwater resources, Earth Syst. Dynam., 5, 15–40, https://doi.org/10.5194/esd-5-15-2014, 2014.
Wadey, M. P., Haigh, Ivan. D., Nicholls, R. J., Brown, J. M., Horsburgh, K., Carroll, B., Gallop, S. L., Mason, T., and Bradshaw, E.: A comparison of the 31 January–1 February 1953 and 5–6 December 2013 coastal flood events around the UK, Front. Mar. Sci., 2, 84, https://doi.org/10.3389/fmars.2015.00084, 2015.
Wahl, T., Jain, S., Bender, J., Meyers, S. D., and Luther, M. E.: Increasing risk of compound flooding from storm surge and rainfall for major US cities, Nat. Clim. Change, 5, 1093–1097, https://doi.org/10.1038/nclimate2736, 2015.
Ward, P. J., Couasnon, A., Eilander, D., Haigh, I. D., Hendry, A., Muis, S., Veldkamp, T. I. E., Winsemius, H. C., and Wahl, T.: Dependence between high sea-level and high river discharge increases flood hazard in global deltas and estuaries, Environ. Res. Lett., 13, 084012, https://doi.org/10.1088/1748-9326/aad400, 2018.
Werner, A. D. and Simmons, C. T.: Impact of Sea-Level Rise on Sea Water Intrusion in Coastal Aquifers, Ground Water, 47, 197–204, https://doi.org/10.1111/j.1745-6584.2008.00535.x, 2009.
Werner, B. T.: Complexity in Natural Landform Patterns, Science, 284, 102–104, https://doi.org/10.1126/science.284.5411.102, 1999.
White, C. J.: The use of joint probability analysis to predict flood frequency in estuaries and tidal rivers, PhD thesis, University of Southampton, 357 pp., 2007.
White, E. and Kaplan, D.: Restore or retreat? saltwater intrusion and water management in coastal wetlands, Ecosystem Health and Sustainability, 3, e01258, https://doi.org/10.1002/ehs2.1258, 2017.
Wicke, B., Smeets, E., Dornburg, V., Vashev, B., Gaiser, T., Turkenburg, W., and Faaij, A.: The global technical and economic potential of bioenergy from salt-affected soils, Energy Environ. Sci., 4, 2669–2681, https://doi.org/10.1039/C1EE01029H, 2011.
Wolff, C., Bonatz, H., and Vafeidis, A. T.: Setback zones can effectively reduce exposure to sea-level rise in Europe, Sci. Rep., 13, 5515, https://doi.org/10.1038/s41598-023-32059-9, 2023.
Wu, W., McInnes, K., O'Grady, J., Hoeke, R., Leonard, M., and Westra, S.: Mapping Dependence Between Extreme Rainfall and Storm Surge, J. Geophys. Res.-Oceans, 123, 2461–2474, https://doi.org/10.1002/2017JC013472, 2018.
Yamazaki, D., Ikeshima, D., Tawatari, R., Yamaguchi, T., O'Loughlin, F., Neal, J. C., Sampson, C. C., Kanae, S., and Bates, P. D.: A high-accuracy map of global terrain elevations: Accurate Global Terrain Elevation map, Geophys. Res. Lett., 44, 5844–5853, https://doi.org/10.1002/2017GL072874, 2017.
Zamrsky, D., Oude Essink, G. H. P., and Bierkens, M. F. P.: Global Impact of Sea Level Rise on Coastal Fresh Groundwater Resources, Earths Future, 12, e2023EF003581, https://doi.org/10.1029/2023EF003581, 2024.
Zscheischler, J., Westra, S., Van Den Hurk, B. J. J. M., Seneviratne, S. I., Ward, P. J., Pitman, A., AghaKouchak, A., Bresch, D. N., Leonard, M., Wahl, T., and Zhang, X.: Future climate risk from compound events, Nat. Clim. Change, 8, 469–477, https://doi.org/10.1038/s41558-018-0156-3, 2018.
Zscheischler, J., Martius, O., Westra, S., Bevacqua, E., Raymond, C., Horton, R. M., Van Den Hurk, B., AghaKouchak, A., Jézéquel, A., Mahecha, M. D., Maraun, D., Ramos, A. M., Ridder, N. N., Thiery, W., and Vignotto, E.: A typology of compound weather and climate events, Nat. Rev. Earth Environ., 1, 333–347, https://doi.org/10.1038/s43017-020-0060-z, 2020.
- Abstract
- Introduction
- Summary of previous assessments relevant to Europe
- The source, pathway, and receptor framework
- Coastal flooding and compounding flood events
- Coastal erosion
- Saltwater intrusion
- Conclusions
- Data availability
- Author contributions
- Competing interests
- Disclaimer
- Acknowledgements
- Financial support
- Review statement
- References
- Abstract
- Introduction
- Summary of previous assessments relevant to Europe
- The source, pathway, and receptor framework
- Coastal flooding and compounding flood events
- Coastal erosion
- Saltwater intrusion
- Conclusions
- Data availability
- Author contributions
- Competing interests
- Disclaimer
- Acknowledgements
- Financial support
- Review statement
- References