the Creative Commons Attribution 4.0 License.
the Creative Commons Attribution 4.0 License.
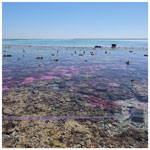
Field experiments in ocean alkalinity enhancement research
Tyler Cyronak
Rebecca Albright
Lennart T. Bach
This chapter focuses on considerations for conducting open-system field experiments in the context of ocean alkalinity enhancement (OAE) research. By conducting experiments in real-world marine and coastal systems, researchers can gain valuable insights into ecological dynamics; biogeochemical cycles; and the safety, efficacy, and scalability of OAE techniques under natural conditions. However, logistical constraints and complex natural dynamics pose challenges. To date, only a limited number of OAE field studies have been conducted, and guidelines for such experiments are still evolving. Due to the fast pace of carbon dioxide removal (CDR) research and development, we advocate for openly sharing data, knowledge, and lessons learned as quickly and efficiently as possible within the broader OAE community and beyond. Considering the potential ecological and societal consequences of field experiments, active engagement with the public and other stakeholders is desirable, while collaboration, data sharing, and transdisciplinary scientific teams can maximize the return on investment. The outcomes of early field experiments are likely to shape the future of OAE research, implementation, and public acceptance, emphasizing the need for transparent and open scientific practices.
- Article
(2385 KB) - Full-text XML
- BibTeX
- EndNote
This chapter addresses considerations for conducting open-system field experiments related to ocean alkalinity enhancement (OAE). We define “field experiment” or “field studies” broadly as the addition or manipulation of alkalinity in a natural system that is relevant to OAE, independent of the spatial and temporal scale. We intentionally exclude spatial and temporal scales from our definition to encompass the wide spectrum of OAE methods and approaches. In fact, field experiments are likely to span spatial scales of squared meters (m2) to hundreds of squared kilometers (km2) and last from days to years. Field experiments and studies differ from both “field trials” and “field deployments” in their motivation, as both trials and deployments denote the practical application and usage of a specific product, device, or technology. The scientific focus during field trials is likely to be on the efficacy of carbon dioxide removal (CDR) and fine-tuning operational deployment, while field experiments will encompass a broader range of scientific goals and objectives. The nature, logistics, and objectives of field experiments are likely to make them smaller in scale than operational deployments. This will be advantageous, as field experiments that emulate planned OAE trials and deployments will help create the scientific framework needed to scale operational OAE safely and responsibly.
The benefits of conducting experiments in natural systems include observing complex ecological dynamics and impacts at the ecosystem level, understanding the role of biogeochemical cycles and physical processes that cannot be replicated in other settings, and assessing CDR under real-world scenarios. The complexity and breadth of some field experiments will necessitate science that transcends disciplinary boundaries, making collaboration a priority. Success in the field faces many challenges due to the inherent complexity of natural systems along with limiting logistical constraints (e.g., permitting, access, social license, infrastructure, life cycle emissions). Despite these challenges, the first OAE field experiments are already underway, many of which are small-scale representations of scalable OAE approaches. There will be much to learn from these early studies, and any knowledge or insights gained should be shared as efficiently and openly as possible within the wider OAE community and beyond.
While some OAE field experiments have been completed or are already in progress, many more are on the horizon. We recommend that three overarching questions be taken into consideration, especially when in the planning stages:
-
What are the main goals of the experiment?
Establishing the objectives of a field experiment early in the planning stage will help guide all aspects of the scientific research plan, including site selection, measurement techniques and approaches, data analysis, and measured outcomes. Potential overarching goals of OAE field experiments include demonstrating functionality, efficacy, process, and/or scalability; determining ecological and environmental impacts; developing measurement, reporting, and verification (MRV) protocols; and assessing community engagement. Life cycle assessments (LCAs) may be a critical learning objective for some projects (e.g., Foteinis et al., 2023), especially those that are examining OAE at the scale of operational deployments. This list of overarching goals is not comprehensive, and goals are not necessarily mutually exclusive. For example, larger projects may aim to assess multiple components of an OAE approach, while smaller projects might be highly focused. -
What is the type of alkalinity perturbation?
The type of alkalinity that is added (e.g., aqueous vs. solid, carbonates, hydroxides, oxides, or naturally occurring (ultra)mafic rocks) will ultimately determine many aspects of the scientific research plan. For example, projects adding ground alkaline minerals (e.g., olivine) to the ocean may have different goals and timelines than projects that add aqueous alkalinity (e.g., liquid NaOH) (see Eisaman et al., 2023, this Guide). Priorities for projects adding ground material might include tracking the dissolution of the alkaline material and monitoring the fate of the dissolved alkalinity and its dissolution coproducts (e.g., trace metals), while projects adding aqueous alkalinity will likely be more concerned with the latter. Other important experimental considerations that will be driven by the type of alkalinity perturbation include the concentration of added alkalinity, duration of additions, dilution and advection at the field site, residence time, air–sea equilibration, co-deployed tracers, sampling scheme, and environmental side effects. These and other research considerations are discussed in more detail below. -
What are the permitting constraints and wider social implications?
Addressing the appropriate regulatory requirements is essential before any field experiment can move forward. Permitting requirements will be influenced by the study location, type of alkalinity perturbation, spatial scale, and duration. The use of existing infrastructure (e.g., wastewater discharge sites) and environmental projects (e.g., beach renourishment) may offer ways to facilitate alkalinity perturbations under existing regulatory frameworks. Community engagement and outreach are other areas that will be important to address, especially when the alkalinity perturbation is large and uncontained. Ideally, local communities should be engaged at the earliest possible stage since social license to operate is critical for the success of CDR projects (Nawaz et al., 2023). For a more detailed discussion of legal and social issues, see Steenkamp and Webb (2023, this Guide) and Satterfield et al. (2023, this Guide).
With these overarching questions in mind, we discuss considerations for OAE field experiments in more detail below.
2.1 Types of alkalinity addition
Field experiments of OAE present many challenges. One of the biggest obstacles to success is tracking alkalinity added to an open system. Methods for adding alkalinity can be divided into two general approaches: (1) in situ or coastal enhanced weathering from the addition of ground alkaline minerals and rocks with the expectation they will dissolve directly in seawater and (2) aqueous alkalinity additions or the addition of “pre-dissolved” alkalinity to seawater that can be generated in numerous ways including through dissolution reactors and electrochemical techniques (Eisaman et al., 2023, this Guide). Tracking the added alkalinity, and subsequent CDR, under each approach comes with its own unique set of challenges and considerations.
Adding ground minerals and rocks to an open system presents two distinct scientific challenges. First, for alkalinity to be considered additional, it needs to be attributed to the dissolution of the solid material. This can be accomplished through a range of techniques including measuring the loss of mass of the added material or using geochemical tracers in the receiving waters. Determining dissolution kinetics in situ will be particularly important, and they are likely to vary between different deployment environments and strategies (e.g., coastal vs. open ocean). For example, the chemistry (e.g., salinity, pH, temperature) of the waters where the mineral is added could vary significantly depending on the environment (e.g., beach face, estuary, continental shelf). Chemical (e.g., seawater conditions, such as salinity, pCO2, and silica concentrations) and physical (e.g., grain size and surface area of the added material) conditions will be critical in determining dissolution rates (Rimstidt et al., 2012; Montserrat et al., 2017; Fuhr et al., 2022). Physical abrasion through wave action and currents is also likely to be an important control on dissolution (Flipkens et al., 2023). Field experiments will help translate dissolution kinetics from laboratory and mesocosm experiments to natural systems, which is not often straightforward due to complicated biogeochemical processes that are hard to replicate ex situ (Morse et al., 2007).
The second major challenge is common to both solid and aqueous approaches and involves tracking the added alkalinity, which becomes a particularly difficult problem in open-system field experiments where water is freely exchanged. Depending on the objectives of the field deployment, this is likely to be a main scientific concern. However, it is important to note that tracking the added alkalinity does not necessarily equate to observing CDR (i.e., an increase in seawater CO2 stored as bicarbonate or carbonate). Observing an increase in atmospheric CO2 stored as seawater dissolved inorganic carbon comes with its own set of challenges that are discussed in depth by Ho et al. (2023, this Guide).
Whether or not the alkalinity is derived from in situ mineral dissolution or direct aqueous additions, for OAE to be successful, atmospheric CO2 needs to be taken up by seawater, or CO2 effluxes from seawater to the atmosphere need to be reduced. Therefore, understanding the physical mixing and air–sea gas exchange dynamics of the deployment site will be a factor of interest for many field studies. Incorporating physical mixing models with biogeochemical processes will likely be the end goal of many field experiments focused on MRV (Ho et al., 2023, this Guide; Fennel et al., 2023, this Guide). Choosing sites with minimal mixing of different water masses or with well-defined diffusivities could facilitate tracing released alkalinity and subsequent air–sea CO2 fluxes. While minimal mixing of different ocean water masses may be desired, higher wind speeds and wave action will increase the rate of air–sea gas exchange and may make CDR easier to measure. Background seawater chemistry will also be important in controlling air–sea gas exchange. For example, sites with naturally lower buffering capacities will see greater changes in CO2 per unit of added alkalinity (Egleston et al., 2010; Hauck et al., 2016). The release of conservative tracers will likely be useful for field experiments that aim to track the added alkalinity and is discussed in more detail below (Sect. 2.5).
Other experimental considerations related to the type of alkalinity perturbation include the duration and location of alkalinity addition, which will be important for environmental and regulatory considerations. Alkalinity can be added once, in timed doses, or continuously. Aqueous alkalinity could be added directly to seawater, but the rate of this addition will likely be important, especially for avoiding secondary precipitation (Hartmann et al., 2023; Moras et al., 2022; Fuhr et al., 2022). Compared to experiments based on one-time additions of aqueous alkalinity or fast-dissolving solid-phase materials (e.g., Ca(OH)2), field experiments adding solid minerals with comparatively slow dissolution rates (e.g., olivine) will likely need to consider longer experimental time frames to incorporate the monitoring of mineral dissolution. However, the timescale of each experiment will ultimately depend on the scientific objectives and could last from weeks to years and even decades. Location is another important factor that will influence logistics. For example, amending beach sand with alkaline minerals will present different challenges compared to the addition of alkaline material to outfalls that discharge into the ocean. Based on these and other considerations, each field experiment will require specific spatial and temporal sampling schemes to be developed. These sampling schemes should be planned well in advance of any perturbation and may require preliminary sampling campaigns to fine tune.
2.2 Alkalinity sources
OAE via coastal enhanced weathering can be accomplished using a variety of naturally occurring and human-made rocks and minerals (Table 1). The addition of these rocks and minerals is done after they have been ground to a desired grain size, with many unique application techniques proposed after the initial grinding step (see Eisaman et al., 2023, this Guide). The simplest application is done via sprinkling the ground material on the ocean surface, although this has many disadvantages including sinking and advection of the material before it dissolves (Köhler et al., 2013; Fakharee et al., 2023), although deployment in boat wakes may be viable (Renforth and Henderson, 2017; He and Tyka, 2023). Other application techniques include spreading material in coastal ecosystems such as on beaches, marshes, riverbeds, and estuaries, which have the potential to enhance dissolution through processes such as physical wave action and favorable water chemistry. However, the complex physical and biogeochemical processes that promote enhanced weathering in coastal ecosystems can make field experimentation more complicated by creating strong spatiotemporal modes of variability in water chemistry. To make results more broadly applicable, field experiments should attempt to mimic real-world alkalinity application scenarios such as those described above.
Any field experiments that add ground material to marine ecosystems may consider tracking the fate of that material from the addition site. Experiments could also artificially contain the material using barriers to avoid rapid loss of the ground material via currents; however, this could make the experiment less comparable to real-world OAE deployments. Sampling should extend from the water column into areas where the material is added, including sediments and pore waters.
Likely environmental impacts associated with coastal enhanced weathering come from the physical impacts of adding finely ground material or the chemical release of trace elements and other contaminants. Both processes could have associated risks and/or co-benefits for a range of ecological processes and biogeochemical cycles (Bach et al., 2019). For example, the addition of finely ground material could lead to increased turbidity from the initial addition, subsequent resuspension, or secondary precipitation of particulates in the water column. Additionally, any release of nutrients or heavy metals from the dissolving material could alter primary production or cause harm to biological systems. The bioaccumulation of toxic metals in higher trophic level organisms, especially those of commercial importance, is a widespread concern.
Safety criteria should be put in place that can create a pause in the field experiment or prevent future experiments of the same type from taking place. These guardrails should be developed by the broader OAE community but may include obvious damage or health impacts to ecologically important organisms such as primary producers and keystone species, large and unexpected changes in biogeochemical cycles, and the general deterioration of environmental conditions. Risk–benefit analysis may be particularly useful in determining whether projects can or should move forward and may already be included in regulatory requirements through existing frameworks such as environmental impact assessments.
Aqueous and slurry-based additions of alkalinity provide different benefits and challenges compared to solid forms of alkalinity feedstock. One of the primary benefits of aqueous additions is that the alkalinity has been pre-dissolved, avoiding the often slow dissolution kinetics of minerals and rocks in seawater. Aqueous alkalinity can be generated by two main mechanisms: (1) the dissolution of alkaline rocks and minerals in reactors and (2) electrochemical processes that generate alkalinity by splitting seawater or other brine streams into an acid and base (Eisaman et al., 2023, this Guide). For some materials, such as Ca(OH)2 and Mg(OH)2, dissolution slurries are formed, and a combination of particulate and aqueous alkalinity can be dosed into seawater. Any particulates that are dosed from the slurry need to dissolve, meaning dissolution kinetics in seawater will be critical. However, the dissolution of these materials tends to be much quicker than with rocks and minerals (Table 1). There are important processes that need to be considered when adding aqueous alkalinity, including the unintended precipitation of calcium carbonates due to locally elevated saturation states (Hartmann et al., 2023; Moras et al., 2022).
Field experiments that use aqueous or slurry-based alkalinity additions will need to assess the impacts on seawater chemistry at the source of addition and across a dilution radius. Depending on the type of experiment and magnitude of additions, this dilution radius could extend upwards of kilometers, but the magnitude of the perturbation to carbonate chemistry would become smaller the further away from the alkalinity source (He and Tyka, 2023). The potential environmental impacts from aqueous type alkalinity additions will be similar to those discussed for coastal enhanced weathering but also include extreme localized changes in carbonate chemistry.
2.3 Considerations for site selection
Careful consideration should be given to site selection and experimental design to make sure the study adequately addresses the specific research questions and goals. Some aspects of the field site that will be important include ecosystem- and site-specific characteristics, the prevailing meteorological and oceanographic conditions, and natural spatiotemporal variability. Logistical considerations for site selection include physical access, permitting, availability of electricity, ship time, and consideration of the local community. These considerations will grow with the scale of field experiments and will likely be first-order determinants of where field experiments take place. For example, proximity to a marine institute (for land-based approaches) or access to a research cruise (for open-ocean approaches) may be desirable. Logistics will ultimately determine where operational OAE deployments take place, and early field experiments will help to elucidate important issues including the impacts of life cycle emissions on CDR.
Table 2Parameters that could be considered in assessing sites for OAE field experiments. Importantly, some parameters summarized below may require a baseline assessment over sufficiently long time frames to cover the intrinsic variability of physical, chemical, and biological parameters in the studied system. For example, baseline assessment of marine food web structure will likely require a prolonged monitoring effort before (and after) the OAE deployment to have a higher chance of detecting OAE-induced effects on marine biota.
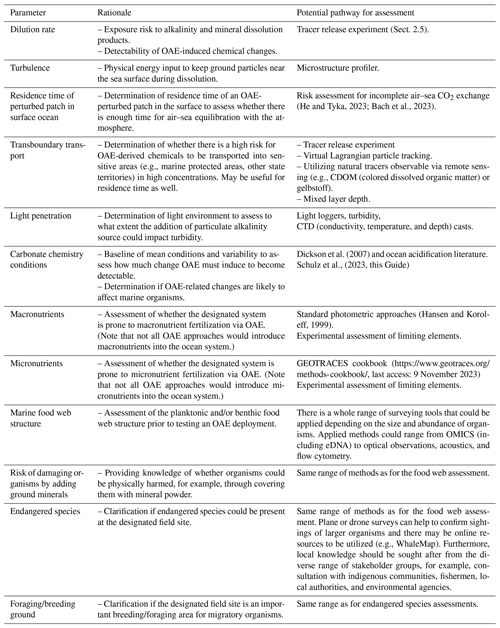
OAE field experimentation requires careful assessment of the field site prior to alkalinity additions to provide foundational knowledge of the site characteristics. Scientific considerations for site selection can be broken down into three categories, the (1) physical, (2) chemical, and (3) biological properties of each site. Important considerations for each category are provided in Box 1. To facilitate baseline assessments and site selection we propose Table 2 as guidance for relevant parameters to measure. We note that this list is broad; however it is not exhaustive, and specific field sites may require the monitoring of different or additional parameters. Furthermore, some of the listed parameters may be more applicable to specific OAE approaches. Preliminary knowledge of the field site will inform both the experimental design and interpretation of data and experimental outcomes. Due to the large investments in cost and time required to collect baseline data, locations with a wealth of pre-existing scientific data may be considered. These baseline data could be available in the peer-reviewed literature and/or from publicly available coastal and open-ocean time series (e.g., Sutton et al., 2019).
2.4 Measurement considerations
What to measure and the type of instrumentation needed will ultimately depend on the site, scale, and goals of each individual experiment and should be considered on a case-by-case basis. For example, depending on the alkalinity source utilized (Table 1), it may (e.g., in the case of olivine) or may not (e.g., in the case of NaOH) be a priority to measure trace metal or nutrient concentrations. In addition to alkalinity type, the experimental scale will also dictate measurement considerations. For example, if the scale of the perturbation is small or the signal is very dilute, environmental impacts will not likely be measurable far from where the perturbation takes place. If there is a large addition of alkalinity, especially in a semi-enclosed system, both environmental impacts and changes in chemistry will be easier to detect. Ultimately, when OAE is done at a larger scale (e.g., millions of moles' alkalinity), it is likely that large changes in seawater chemistry will need to be avoided to reduce environmental impacts and avoid secondary precipitation. This presents an interesting challenge to conducting field experiments, as the dilution of alkalinity and ultimately CO2 signal will make MRV more challenging (Ho et al., 2023, this Guide).
Seawater carbonate chemistry measurements will be central to most sampling schemes. To cover the appropriate spatial and temporal scales, traditional bottle sampling will likely have to be combined with state-of-the-art in situ sensors (Bushinsky et al., 2019; Briggs et al., 2020; Ho et al., 2023, this Guide). Bushinsky et al. (2019; their Fig. 1) provide a comprehensive overview of the spatiotemporal capabilities of existing carbonate chemistry sensors and platforms, and care should be taken to make sure sensors are appropriate for measurements in seawater. The appropriate methods and protocols for sampling and analysis are outlined in other chapters in this guide (Schulz et al., 2023, this Guide) and in the Guide to Best Practices (Dickson et al., 2007). Some general considerations for field experiments include appropriately characterizing the natural variability that occurs at the field site through space and time. While total alkalinity (TA) titrations should remain a priority, at least two carbonate chemistry parameters (e.g., total alkalinity, dissolved inorganic carbon, pH, or pCO2) should be measured for each sample. It is important to note that the combination of pCO2 and pH is not ideal when calculating CO2 chemistry (e.g., using CO2SYS) due to the elevated errors when combining those parameters in determining the rest of the carbonate chemistry system in seawater (Lee and Millero, 1995). Currently, commercially available autonomous sensors exist for pH and pCO2, with sensors in development for both TA and dissolve inorganic carbon (DIC; Fassbender et al., 2015; Briggs et al., 2020; Qiu et al., 2023). While autonomous sensors generally have greater uncertainty than bottle samples coupled with laboratory analysis, they will likely play an important role in sampling schemes to help cover adequate spatial and temporal resolution in naturally variable marine systems.
While monitoring the background variability and subsequent additions of alkalinity will be critical, scientists may also wish to directly measure fluxes of carbon at the field study site (Ho et al., 2023, this Guide). The direct measurement of carbon fluxes can be accomplished via different methods including benthic and floating chambers, eddy covariance and other benthic boundary layer techniques, and mass balances. These techniques have benefits and drawbacks, including having to enclose the natural system (e.g., chambers) and elevated uncertainty that could be outside of the expected changes due to the perturbation (e.g., eddy covariance). Benthic chamber measurements may be particularly important to quantify the dissolution of minerals and rocks added to sediments. Ultimately, any measurements of fluxes due to OAE activities will likely need to be coupled with numerical modeling to estimate the overall drawdown of atmospheric CO2 (Fennel et al., 2023, this Guide).
Field experiments should be informed by other scientific studies as much as possible (e.g., studies based on laboratory experiments, mesocosm studies, natural analogs, and numerical modeling). While not necessarily directly translatable to natural systems (Edmunds et al., 2016; Page et al., 2022), these types of studies can provide first-order assessments on safety and efficacy, helping to prevent unintended harmful ecological side effects when conducting large-scale perturbations.
Other measurements that may be useful during OAE field experiments are outlined in Table 2. It is important to note that this list is not meant to be exhaustive, and measurement selection will have to be made on a case-by-case basis. Considering the difficulties of tracking water masses in an open system, the next section is a more detailed discussion on tracers for monitoring mixing and dilution of water within the OAE field experiment site. Tracking added alkalinity will be critical to determine the impacts and efficacy of alkalinity enrichments and may be one of the biggest challenges facing OAE field experiments.
2.5 Dual-tracer regression technique
If the goal is to track alkalinity additions and measure their effects on carbon fluxes (i.e., net ecosystem production or air–sea exchange), a dual-tracer regression method can be used (e.g., Albright et al., 2016, 2018). This approach uses the change in ratios between an active tracer (alkalinity) and a passive tracer (dye, artificial gas tracer; Table 3) to assess the fraction of added alkalinity taken up or released by biogeochemical processes in the system. Passive tracers do not affect fluid dynamics and are passively advected by the surrounding flow field. The use of passive tracers, such as dye tracers (e.g., rhodamine, fluorescein) or artificial gas tracers (e.g., SF6, CF3SF5), that do not occur in nature helps eliminate background noise. Additional considerations include how many tracers to use and what information each tracer provides (Table 3).
Table 3Passive tracers that are available and commonly used for use in field experiments and considerations for each. Additional tracers may be useful that are not listed in this table, including helium 3 and tritium.
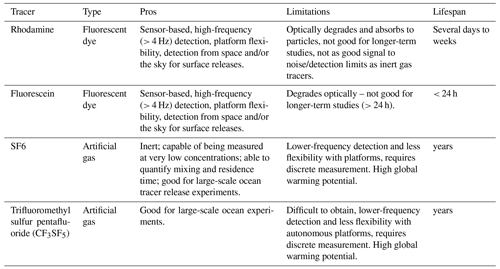
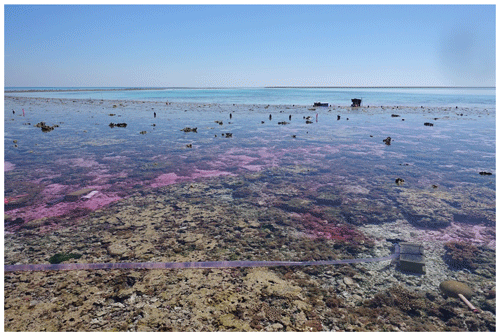
Figure 1Rhodamine dye flowing over a coral reef flat study site during a study in One Tree Island, Australia (Albright et al., 2016). NaOH was used as an active tracer to raise alkalinity, and rhodamine was used as a passive tracer to account for mixing and dilution. Changes in the alkalinity-to-dye ratios were used to isolate the change in alkalinity flux that was associated with an increase in net community calcification on the reef flat.
During a dual-tracer experiment, changes in the active tracer (alkalinity) result from mixing, dilution, and biogeochemical activity, whereas changes in the passive tracer are due solely to mixing and dilution. By comparing the alkalinity-to-dye ratios before (e.g., upstream) and after (e.g., downstream) the water mass interacts with a study area, it is possible to isolate the change in alkalinity that is due to biogeochemical processes such as calcium carbonate precipitation and dissolution (Figs. 1 and 2). This technique is an extension of Friedlander et al. (1986) and may have applications in other areas of research pertinent to marine CDR, such as nutrient or pollution assessments and the uptake of industrial or agricultural waste. The primary experimental criteria for the dual-tracer technique are that the active and passive tracers are added in a fixed ratio and at a fixed rate, in areas where there is a dominant flow direction, dispersion, or dilution.
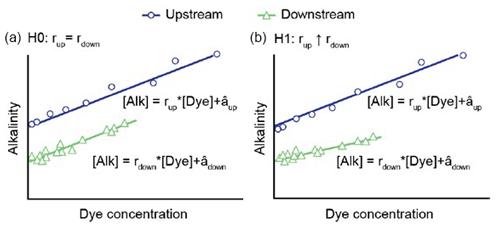
Figure 2Theoretical representations of the null (H0) and alternative (H1) hypotheses for a dual-tracer regression experiment where NaOH was used as a source of alkalinity and rhodamine dye was used as a passive tracer (from Albright et al., 2016). (a) In H0, the benthic community does not take up added alkalinity. Here, the change in alkalinity between the upstream and downstream transects would not be systematically related to the dye concentration, and the ratio of the alkalinity–dye relationship, r, would not be expected to change between the upstream and downstream locations (that is, rup=rdown). (b) In H1, an uptake of added alkalinity occurs by the benthic community. Here, areas with more alkalinity (and more dye) change at a different rate than areas with less alkalinity (and less dye), resulting in a change in the alkalinity–dye slope (that is, rup > rdown).
2.6 Detecting change and the importance of controlled experiments
Separating an experimental “signal” from the background “noise” inherent in natural systems can be challenging, especially in field experiments where replication may not be practical (Carpenter, 1990). Gaining baseline knowledge on the physical, chemical, and biological components of the study site should be a priority. There is often considerable natural variability in marine systems, and especially in coastal systems, due to fluctuations in biological activity, hydrodynamics, seasonal and/or interannual influences, and other factors (Bates et al., 1998; Bates, 2002; Hagens and Middelburg, 2016; Landschützer et al., 2018; Sutton et al., 2019; Kapsenberg and Cyronak, 2019; Torres et al., 2021). Fully characterizing this variability could take many years, which may create significant barriers to experimental progress in the field. Therefore, we recommend that any potential modes of spatiotemporal variability be recognized and evaluated while planning field experiments. For instance, in coastal systems with river and groundwater inputs, it will be important to know the impact that freshwater has on carbonate chemistry.
Where possible, conducting controlled experiments will help to maximize the ratio of signal to noise, thereby improving statistical power to detect experimental effects. The pros and cons of replicating experimental controls in space versus time should be taken into consideration. For many field experiments (and natural analogs; see Subhas et al., 2023, this Guide), sample size will be inherently limited (e.g., one, or few study sites); therefore, conducting controls in time (e.g., every third day) may be the best option. For studies with limited (or no) replication, there are statistical methods that can be used to isolate effects pre- and post-treatment (Carpenter, 1990). Numerical simulations and machine-learning-based network design are potentially valuable tools to optimize observational networks to detect experimental change.
Permitting. Addressing regulatory requirements is critical prior to conducting field experiments. The spatial and temporal scale of the field trial, as well as the specific considerations of the deployment site (e.g., protection status), will determine permitting requirements. Engaging with this process early is advised – for example, understanding who the permit-granting authorities are for a given area and timelines for associated regulatory processes. In some cases, the use of existing infrastructure (e.g., wastewater discharge sites) and environmental projects (e.g., beach renourishment) may offer ways to streamline experiments, although permitting will be governed by existing regulations. For a detailed discussion on legal considerations, see Steenkamp and Webb (2023, this Guide).
Community engagement and social considerations of field experiments. The likelihood of harmful ecological consequences from OAE field experiments remains unclear and will ultimately depend on the technology and temporal and spatial scale of the experiment. Field experiments evaluating CDR approaches carry the risk of unintended consequences and impacts over large spatial scales, so appropriate scaling (e.g., starting small) is necessary (NASEM, 2022). In response to these unknowns, researchers should follow the key components for a code of conduct for marine CDR research, e.g., as outlined by Loomis et al. (2022), which details best practices that encourage responsible research amongst both the public and private sectors.
Social license to operate is critical for the success of CDR projects, and researchers have an obligation to involve the full community of people (public and stakeholders) who may be impacted by the research (Nawaz et al., 2023; Cooley et al., 2023). Therefore, public outreach is important both before and during field experimentation. The study site will determine the potential for community engagement. Coordinating with local and/or regional organizations who are connected to relevant stakeholders (for example, your local SeaGrant office if in the United States) will be helpful. For additional discussion on social considerations of OAE field trials, see Satterfield et al. (2023, this Guide).
Collaboration and data/information sharing. Considering the inherent challenges to OAE field experiments (cost, permitting, access, logistics, environmental safety), fostering interdisciplinary and collaborative teams will help ensure the greatest return on investment. Examples of ways to foster collaboration include developing test-bed field sites that are open to participation from diverse stakeholder groups (https://oceanvisions.org/highlevelroadmap/, last access: 14 November 2023), making efforts to include groups who may not traditionally have access to and/or the capacity for field campaigns, and including travel support in grant applications to support external collaborators. Making concerted efforts to share information, resources, and ideas will allow researchers to combine knowledge and resources in ways that might not have been possible when working alone, thereby advancing OAE technology and science at a faster pace. When publishing in peer-reviewed literature, uploading data to publicly available data repositories and publishing in open-access journals following best practices should be prioritized (Jiang et al., 2023, this Guide).
Inclusivity and transparency during OAE field trials are crucial to ensure that knowledge gained is fed back into scientific and other communities efficiently, iteratively informing and refining the next generation of experiments. Some field experiments will mimic plans for real-world OAE deployments and should therefore be done in collaboration with relevant stakeholders across science, industry, policy, and communities. To foster collaboration and technology transfer, we advocate for a centralized platform and/or organization to share data and information in this rapidly evolving field. This might look like a centralized, freely accessible platform for early and/or “real-time” information sharing (i.e., before publication) that can facilitate faster information exchange within the research community (e.g., data sharing, permitting issues). Two existing options that could help fill this gap are the Ocean Acidification Information Exchange (https://www.oainfoexchange.org/index.html, last access: 11 November 2023) and the Ocean Visions community (https://community.oceanvisions.org/dashboard, last access: 11 November 2023). It may prove useful to designate core working groups of experts in various aspects of CDR that investigate specific needs and priorities and work to synthesize and share existing knowledge in the context of field experiments. This approach has been adopted by other scientific disciplines in high-priority, rapidly evolving, and highly collaborative fields, greatly benefiting the scientific community at large (e.g., the Coral Restoration Consortium, https://www.crc.world/, last access: 11 November 2023 – and associated working groups). Coordinating field trials with research groups conducting laboratory and mesocosm experiments, studying natural analogs, and undertaking modeling efforts will help strengthen the interpretation and extrapolation of results.
Given that few OAE field studies have been conducted to date, there is much to learn from the earliest experiments with respect to experimental design, measurement and monitoring, deployment considerations, environmental impact, and more. Early experiments will only engage with a fraction of the temporal and spatial scales involved in full-scale operational OAE, and longer-term and larger-scale studies will become increasingly important to reveal scale dependencies as the field develops. It is important that marine CDR research is hypothesis-driven, structured, deliberate, and well-planned to best inform future decision-making about OAE techniques and deployments. Careful consideration of the physical, chemical, and biological components of the study area will help inform the experimental approach. The use of baseline studies (both previous and contemporary to the OAE deployment) and controls will help to maximize signal-to-noise ratios and identify experimental effects. The timescale of OAE field experiments should not be underestimated, especially when considering permitting, and the data needed to capture the baseline variability in natural systems.
Considering the urgent timeline required for humanity to meet our climate goals, field experiments need to move forward swiftly yet deliberately. To ensure the success of OAE, diverse perspectives from research, industry, policy, and society must converge, demanding transdisciplinary thinking and a commitment to open and transparent science. Central to this ambitious undertaking are the early field experiments, results from which will ultimately determine the successes and failures of OAE projects and technologies.
Key recommendations
-
Ensure inclusivity and transparency (community engagement, data sharing, etc.) for OAE field experiments to both advance the field as quickly as possible and ensure the field progresses in a socially responsible manner.
-
Assess the potential risks and benefits for any perturbation. Proceed according to a code of conduct and precautionary principles.
-
Develop methods to track signal versus noise in highly variable environments, including robust baseline studies to characterize underlying variability (biological, chemical, physical), and include controlled experiments such as chamber incubations to isolate treatment effects.
-
Consider the logistical constraints and opportunities of field locations.
-
Create test-bed field sites that are open to participation from diverse stakeholder groups.
No data sets were used in this article.
TC, RA, and LTB all contributed to the conceptualization and writing of this paper.
Competing interests are declared in a summary for the entire volume at: https://sp.copernicus.org/articles/sp-oae2023-ci-summary.zip.
Publisher’s note: Copernicus Publications remains neutral with regard to jurisdictional claims made in the text, published maps, institutional affiliations, or any other geographical representation in this paper. While Copernicus Publications makes every effort to include appropriate place names, the final responsibility lies with the authors.
We thank the Ocean Acidification and other ocean Changes – Impacts and Solutions (OACIS), an initiative of the Prince Albert II of Monaco Foundation, for its support throughout the project. We extend our gratitude to the Villefranche Oceanographic Laboratory for supporting the meeting of the lead authors in January 2023. Tyler Cyronak was supported by the Tides Foundation through a Google Carbon Removal Research Award. Lennart T. Bach was supported by the Australian Research Council through Future Fellowship (FT200100846) and by the Carbon-to-Sea Initiative.
This research has been supported by the ClimateWorks Foundation (grant no. 22-0296) and the Prince Albert II of Monaco Foundation. It has also been supported by the Tides Foundation through a Google Carbon Removal Research Award, the Australian Research Council through Future Fellowship (grant no. FT200100846), and the Carbon-to-Sea Initiative.
This paper was edited by Romany Webb and reviewed by Will Burt, Lester Kwiatkowski, M. Grace Andrews, and Alex Poulton.
Albright, R., Caldeira, L., Hosfelt, J., Kwiatkowski, L., Maclaren, J. K., Mason, B. M., Nebuchina, Y., Ninokawa, A., Pongratz, J., Ricke, K. L., Rivlin, T., Schneider, K., Sesboue, M., Shamberger, K., Silverman, J., Wolfe, K., Zhu, K., and Caldeira, K.: Reversal of ocean acidification enhances net coral reef calcification, Nature, 531, 362–365, 2016.
Albright, R., Takeshita, Y., Koweek, D. A., Ninokawa, A., Wolfe, K., Rivlin, T., Nebuchina, Y., Young, J., and Caldeira, K.: Carbon dioxide addition to coral reef waters suppresses net community calcification, Nature, 555, 516–519, 2018.
Bach, L. T., Gill, S. J., Rickaby, R. E., Gore, S., and Renforth, P.: CO2 removal with enhanced weathering and ocean alkalinity enhancement: potential risks and co-benefits for marine pelagic ecosystems, Frontiers in Climate, 1, 7, https://doi.org/10.3389/fclim.2019.00007, 2019.
Bach, L. T., Ho, D. T., Boyd, P. W., and Tyka, M. D.: Toward a consensus framework to evaluate air–sea CO2 equilibration for marine CO2 removal, Limnol. Oceanogr. Lett., 8, 685–691, https://doi.org/10.1002/lol2.10330, 2023.
Bates, N. R.: Seasonal variability of the effect of coral reefs on seawater CO2 and air–sea CO2 exchange, Limnol. Oceanogr., 47, 43–52, 2002.
Bates, N. R., Takahashi, T., Chipman, D. W., and Knap, A. H.: Variability of pCO2 on diel to seasonal timescales in the Sargasso Sea near Bermuda, J. Geophys. Res.-Oceans, 103, 15567–15585, 1998.
Briggs, E. M., De Carlo, E. H., Sabine, C. L., Howins, N. M., and Martz, T. R.: Autonomous ion-sensitive field effect transistor-based total alkalinity and pH measurements on a barrier reef of Kane'ohe Bay, ACS Earth and Space Chemistry, 4, 355–362, 2020.
Bushinsky, S. M., Takeshita, Y., and Williams, N. L.: Observing changes in ocean carbonate chemistry: our autonomous future, Current Climate Change Reports, 5, 207–220, 2019.
Carpenter, S. R.: Large-scale perturbations: opportunities for innovation, Ecology, 71, 2038–2043, 1990.
Cooley, S. R., Klinsky, S., Morrow, D. R., and Satterfield, T.: Sociotechnical considerations about ocean carbon dioxide removal, Annu. Rev. Mar. Sci., 15, 41–66, 2023.
Dickson, A. G., Sabine, C. L., and Christian, J. R. (Eds.): Guide to best practices for ocean CO2 measurements, North Pacific Marine Science Organization, Sidney, British Columbia, PICES Special Publication 3, 191 pp., https://doi.org/10.25607/OBP-1342, 2007.
Edmunds, P. J., Comeau, S., Lantz, C., Andersson, A., Briggs, C., Cohen, A., Gattuso, J.-P., Grady, J. M., Gross, K., Johnson, M., Muller, E. B., Ries, J. B., Tambutte, S., Tambutte, E., Venn, A., and Carpenter, R. C.: Integrating the effects of ocean acidification across functional scales on tropical coral reefs, Bioscience, 66, 350–362, 2016.
Egleston, E. S., Sabine, C. L., and Morel, F. M. M.: Revelle revisited: Buffer factors that quantify the response of ocean chemistry to changes in DIC and alkalinity, Global Biogeochem. Cy., 24, GB1002, https://doi.org/10.1029/2008GB003407, 2010.
Eisaman, M. D., Geilert, S., Renforth, P., Bastianini, L., Campbell, J., Dale, A. W., Foteinis, S., Grasse, P., Hawrot, O., Löscher, C. R., Rau, G. H., and Rønning, J.: Assessing the technical aspects of ocean-alkalinity-enhancement approaches, in: Guide to Best Practices in Ocean Alkalinity Enhancement Research, edited by: Oschlies, A., Stevenson, A., Bach, L. T., Fennel, K., Rickaby, R. E. M., Satterfield, T., Webb, R., and Gattuso, J.-P., Copernicus Publications, State Planet, 2-oae2023, 3, https://doi.org/10.5194/sp-2-oae2023-3-2023, 2023.
Fakhraee, M., Li, Z., Planavsky, N. J., and Reinhard, C. T.: A biogeochemical model of mineral-based ocean alkalinity enhancement: impacts on the biological pump and ocean carbon uptake, Environ. Res. Lett., 18, 044047, https://doi.org/10.1088/1748-9326/acc9d4, 2023.
Fassbender, A. J., Sabine, C. L., Lawrence-Slavas, N., De Carlo, E. H., Meinig, C., and Maenner Jones, S.: Robust sensor for extended autonomous measurements of surface ocean dissolved inorganic carbon, Environ. Sci. Technol., 49, 3628–3635, 2015.
Fennel, K., Long, M. C., Algar, C., Carter, B., Keller, D., Laurent, A., Mattern, J. P., Musgrave, R., Oschlies, A., Ostiguy, J., Palter, J. B., and Whitt, D. B.: Modelling considerations for research on ocean alkalinity enhancement (OAE), in: Guide to Best Practices in Ocean Alkalinity Enhancement Research, edited by: Oschlies, A., Stevenson, A., Bach, L. T., Fennel, K., Rickaby, R. E. M., Satterfield, T., Webb, R., and Gattuso, J.-P., Copernicus Publications, State Planet, 2-oae2023, 9, https://doi.org/10.5194/sp-2-oae2023-9-2023, 2023.
Flipkens, G., Fuhr, M., Fiers, G., Meysman, F. J. R., Town, R. M., and Blust, R.: Enhanced olivine dissolution in seawater through continuous grain collisions, Geochim. Cosmochim. Ac., 359, 84–99, 2023.
Foteinis, S., Campbell, J. S., and Renforth P.: Life Cycle Assessment of Coastal Enhanced Weathering for Carbon Dioxide Removal from Air, Environ. Sci. Technol., 57, 6169–6178, 2023.
Friedlander, S. K., Turner, J. R., and Hering, S. V.: A new method for estimating dry deposition velocities for atmospheric aerosols, J. Aerosol Sci., 17, 240–244, 1986.
Fuhr, M., Geilert, S., Schmidt, M., Liebetrau, V., Vogt, C., Ledwig, B., and Wallmann, K.: Kinetics of Olivine Weathering in Seawater: An Experimental Study, Frontiers in Climate, 4, 831587, https://doi.org/10.3389/fclim.2022.831587, 2022.
Hagens, M. and Middelburg, J. J.: Attributing seasonal pH variability in surface ocean waters to governing factors, Geophys. Res. Lett., 43, 12528–12537, https://doi.org/10.1002/2016GL071719, 2016.
Hansen, H. P. and Koroleff, F.: Determination of nutrients, in: Methods of seawater analysis, edited by: Grasshoff, K., Kremling, K., and Ehrhardt, M., 159–228, https://doi.org/10.1002/9783527613984.ch10, 1999.
Hartmann, J., Suitner, N., Lim, C., Schneider, J., Marín-Samper, L., Arístegui, J., Renforth, P., Taucher, J., and Riebesell, U.: Stability of alkalinity in ocean alkalinity enhancement (OAE) approaches – consequences for durability of CO2 storage, Biogeosciences, 20, 781–802, https://doi.org/10.5194/bg-20-781-2023, 2023.
Hauck, J., Köhler, P., Wolf-Gladrow, D., and Völker, C.: Iron fertilisation and century-scale effects of open ocean dissolution of olivine in a simulated CO2 removal experiment, Environ. Res. Lett., 11, 024007, https://doi.org/10.1088/1748-9326/11/2/024007, 2016.
He, J. and Tyka, M. D.: Limits and CO2 equilibration of near-coast alkalinity enhancement, Biogeosciences, 20, 27–43, https://doi.org/10.5194/bg-20-27-2023, 2023.
Ho, D. T., Bopp, L., Palter, J. B., Long, M. C., Boyd, P. W., Neukermans, G., and Bach, L. T.: Monitoring, reporting, and verification for ocean alkalinity enhancement, in: Guide to Best Practices in Ocean Alkalinity Enhancement Research, edited by: Oschlies, A., Stevenson, A., Bach, L. T., Fennel, K., Rickaby, R. E. M., Satterfield, T., Webb, R., and Gattuso, J.-P., Copernicus Publications, State Planet, 2-oae2023, 12, https://doi.org/10.5194/sp-2-oae2023-12-2023, 2023.
Jiang, L.-Q., Subhas, A. V., Basso, D., Fennel, K., and Gattuso, J.-P.: Data reporting and sharing for ocean alkalinity enhancement research, in: Guide to Best Practices in Ocean Alkalinity Enhancement Research, edited by: Oschlies, A., Stevenson, A., Bach, L. T., Fennel, K., Rickaby, R. E. M., Satterfield, T., Webb, R., and Gattuso, J.-P., Copernicus Publications, State Planet, 2-oae2023, 13, https://doi.org/10.5194/sp-2-oae2023-13-2023, 2023.
Kapsenberg, L. and Cyronak, T.: Ocean acidification refugia in variable environments, Glob. Change Biol., 25, 3201–3214, 2019.
Köhler, P., Abrams, J. F., Völker, C., Hauck, J., and Wolf-Gladrow, D. A.: Geoengineering impact of open ocean dissolution of olivine on atmospheric CO2, surface ocean pH and marine biology, Environ. Res. Lett., 8, 014009, https://doi.org/10.1088/1748-9326/8/1/014009, 2013.
Landschützer, P., Gruber, N., Bakker, D. C. E., Stemmler, I., and Six, K. D.: Strengthening seasonal marine CO2 variations due to increasing atmospheric CO2, Nat. Clim. Change, 8, 146–150, 2018.
Lee, K. and Millero, F. J.: Thermodynamic studies of the carbonate system in seawater, Deep-Sea Res. Pt. I, 42, 2035–2061, 1995.
Loomis, R., Cooley, S. R., Collins, J. R., Engler, S., and Suatoni, L.: A Code of Conduct Is Imperative for Ocean Carbon Dioxide Removal Research, Frontiers in Marine Science, 9, 872800, https://doi.org/10.3389/fmars.2022.872800, 2022.
Montserrat, F., Renforth, P., Hartmann, J., Leermakers, M., Knops, P., and Meysman, F. J. R.: Olivine Dissolution in Seawater: Implications for CO2 Sequestration through Enhanced Weathering in Coastal Environments, Environ. Sci. Technol., 51, 3960–3972, 2017.
Moras, C. A., Bach, L. T., Cyronak, T., Joannes-Boyau, R., and Schulz, K. G.: Ocean alkalinity enhancement – avoiding runaway CaCO3 precipitation during quick and hydrated lime dissolution, Biogeosciences, 19, 3537–3557, https://doi.org/10.5194/bg-19-3537-2022, 2022.
Morse, J. W., Arvidson, R. S., and Lüttge, A.: Calcium carbonate formation and dissolution, Chem. Rev., 107, 342–381, 2007.
National Academies of Sciences, Engineering, and Medicine (NASEM): A Research Strategy for Ocean-based Carbon Dioxide Removal and Sequestration, The National Academies Press, Washington, DC, https://doi.org/10.17226/26278, 2022.
Nawaz, S., Peterson St-Laurent, G., and Satterfield, T.: Public evaluations of four approaches to ocean-based carbon dioxide removal, Clim. Policy, 23, 379–394, 2023.
Page, H. N., Bahr, K. D., Cyronak, T., Jewett, E. B., Johnson, M. D., and McCoy, S. J.: Responses of benthic calcifying algae to ocean acidification differ between laboratory and field settings, ICES J. Mar. Sci., 79, 1–11, 2022.
Qiu, L., Li, Q., Yuan, D., Chen, J., Xie, J., Jiang, K., Guo, L., Zhong, G., Yang, B., and Achterberg, E. P.: High-Precision In Situ Total Alkalinity Analyzer Capable of Month-Long Observations in Seawaters, ACS Sensors, 8, 2702–2712, 2023.
Renforth, P. and Henderson, G.: Assessing ocean alkalinity for carbon sequestration, Rev. Geophys., 55, 636–674, 2017.
Rimstidt, J. D., Brantley, S. L., and Olsen, A. A.: Systematic review of forsterite dissolution rate data, Geochim. Cosmochim. Ac., 99, 159–178, 2012.
Satterfield, T., Nawaz, S., and Boettcher, M.: Social considerations and best practices to apply to engaging publics on ocean alkalinity enhancement, in: Guide to Best Practices in Ocean Alkalinity Enhancement Research, edited by: Oschlies, A., Stevenson, A., Bach, L. T., Fennel, K., Rickaby, R. E. M., Satterfield, T., Webb, R., and Gattuso, J.-P., Copernicus Publications, State Planet, 2-oae2023, 11, https://doi.org/10.5194/sp-2-oae2023-11-2023, 2023.
Schulz, K. G., Bach, L. T., and Dickson, A. G.: Seawater carbonate chemistry considerations for ocean alkalinity enhancement research: theory, measurements, and calculations, in: Guide to Best Practices in Ocean Alkalinity Enhancement Research, edited by: Oschlies, A., Stevenson, A., Bach, L. T., Fennel, K., Rickaby, R. E. M., Satterfield, T., Webb, R., and Gattuso, J.-P., Copernicus Publications, State Planet, 2-oae2023, 2, https://doi.org/10.5194/sp-2-oae2023-2-2023, 2023.
Steenkamp, R. C. and Webb, R.: Legal considerations relevant to research on ocean alkalinity enhancement, in: Guide to Best Practices in Ocean Alkalinity Enhancement Research, edited by: Oschlies, A., Stevenson, A., Bach, L. T., Fennel, K., Rickaby, R. E. M., Satterfield, T., Webb, R., and Gattuso, J.-P., Copernicus Publications, State Planet, 2-oae2023, 10, https://doi.org/10.5194/sp-2-oae2023-10-2023, 2023.
Subhas, A. V., Lehmann, N., and Rickaby, R. E. M.: Natural analogs to ocean alkalinity enhancement, in: Guide to Best Practices in Ocean Alkalinity Enhancement Research, edited by: Oschlies, A., Stevenson, A., Bach, L. T., Fennel, K., Rickaby, R. E. M., Satterfield, T., Webb, R., and Gattuso, J.-P., Copernicus Publications, State Planet, 2-oae2023, 8, https://doi.org/10.5194/sp-2-oae2023-8-2023, 2023.
Sutton, A. J., Feely, R. A., Maenner-Jones, S., Musielwicz, S., Osborne, J., Dietrich, C., Monacci, N., Cross, J., Bott, R., Kozyr, A., Andersson, A. J., Bates, N. R., Cai, W.-J., Cronin, M. F., De Carlo, E. H., Hales, B., Howden, S. D., Lee, C. M., Manzello, D. P., McPhaden, M. J., Meléndez, M., Mickett, J. B., Newton, J. A., Noakes, S. E., Noh, J. H., Olafsdottir, S. R., Salisbury, J. E., Send, U., Trull, T. W., Vandemark, D. C., and Weller, R. A.: Autonomous seawater pCO2 and pH time series from 40 surface buoys and the emergence of anthropogenic trends, Earth Syst. Sci. Data, 11, 421–439, https://doi.org/10.5194/essd-11-421-2019, 2019.
Torres, O., Kwiatkowski, L., Sutton, A. J., Dorey, N., and Orr, J. C.: Characterizing Mean and Extreme Diurnal Variability of Ocean CO2 System Variables Across Marine Environments, Geophys. Res. Lett., 48, e2020GL090228, https://doi.org/10.1029/2020GL090228, 2021.