the Creative Commons Attribution 4.0 License.
the Creative Commons Attribution 4.0 License.
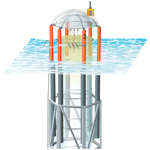
Mesocosm experiments in ocean alkalinity enhancement research
Daniela Basso
Sonja Geilert
Andrew W. Dale
Matthias Kreuzburg
An essential prerequisite for the implementation of ocean alkalinity enhancement (OAE) applications is their environmental safety. Only if it can be ensured that ecosystem health and ecosystem services are not at risk will the implementation of OAE move forward. Public opinion on OAE strategies will depend first and foremost on reliable evidence that no harm will be done to marine ecosystems, and licensing authorities will demand measurable criteria against which environmental sustainability can be determined. In this context mesocosm experiments represent a highly valuable tool in determining the safe operating space of OAE applications. By combining biological complexity with controllability and replication, they provide an ideal OAE test bed and a critical stepping stone towards field applications. Mesocosm approaches can also be helpful in testing the efficacy, efficiency and permanence of OAE applications. This chapter outlines strengths and weaknesses of mesocosm approaches, illustrates mesocosm facilities and suitable experimental designs presently employed in OAE research, describes critical steps in mesocosm operation, and discusses possible approaches for alkalinity manipulation and monitoring. Building on a general treatise on each of these aspects, the chapter describes pelagic and benthic mesocosm approaches separately, given their inherent differences. The chapter concludes with recommendations for best practices in OAE-related mesocosm research.
- Article
(8373 KB) - Full-text XML
- BibTeX
- EndNote
The authors would like to emphasize that this chapter does not intend to cover all aspects of mesocosm experimentation in its full breadth, but rather it tries to address aspects specific to research on ocean alkalinity enhancement (OAE) or aspects we consider important to reiterate here. For a more comprehensive presentation of recommendations and guidelines on mesocosm experiments the reader is referred to Chapter 6 of the Guide for Best Practices on Ocean Acidification Research and Data Reporting (Riebesell et al., 2010) and references therein as well as Stewart et al. (2013).
Although the general approach to mesocosm experiments is straightforward and basically involves enclosing a body of water with or without sediment in order to monitor responses of the enclosed communities and related processes to the manipulated perturbation over an extended period of time, the specifics of conducting such experiments can vary considerably. These include factors such as the materials, design and location of the enclosures (e.g., fixed structures on land or flexible wall enclosures in situ) as well as the procedures for mesocosm filling, operation, mixing and sampling. While the dimensions of the experimental enclosures can range from less than 1 m3 to > 1000 m3 depending on the requirements of the experiment, we here adopt the classification set out by the SCOR Working Group 85 (1991): microcosms (less than 1 m3), mesocosms (between 1 and 1000 m3) and macrocosms (more than 1000 m3). We note that benthic experimental enclosures can have different size categories.
Mesocosm experiments provide an essential bridge between the tightly controlled but poorly realistic laboratory culture experiments and the complexity of natural systems. This is particularly important for possible OAE implementations, in order to achieve a sound understanding of the entire process of the proposed OAE strategies, from the dissolution kinetics and effectiveness of the alkalinization technique to the potential environmental impacts, risks and co-benefits. This knowledge is crucial prior to any form of OAE application to safeguard the protection of marine ecosystems functioning, biodiversity and related ecosystem services. Moreover, should OAE prove to be a viable approach for marine carbon dioxide removal (mCDR), it will also be crucial to achieve social acceptance for potential OAE implementations. Also in this context mesocosm experiments can serve as a useful tool for proof of concept, the results of which can play an important role in the public discourse about the risks and benefits of mCDR implementation.
Functional redundancy and species richness in ecosystems allow for some degree of resistance to withstand disturbances and resilience to recover once a disturbance has ended or dissipated. To determine the actual ecological impacts of OAE it is essential, therefore, to test suggested applications at the community/ecosystem level. Doing this in field trials, however, poses serious difficulties, given the hydrographic complexity of most marine systems, with lateral advection (currents, tides), vertical flow (convection, up- and downwelling) and wave-driven mixing. Determining dose–response relationships for environmental impacts is extremely challenging under such conditions. Mesocosm experiments, on the other hand, enable the combination of biological complexity needed for testing resistance and resilience of communities/ecosystems in their natural setting and seasonal succession (in a single experiment where succession occurs on short timescales, e.g., a phytoplankton bloom, or multiple experiments in different seasons using the exact same experimental setup) with a reasonable degree of control and replication and hence the statistical power to reach reliable conclusions. At the same time, they allow testing the chemical kinetics of mineral dissolution and secondary carbonate precipitation, thereby providing vital information on the efficacy of the suggested OAE applications in a natural setting under a range of environmental conditions (salinity, temperature, carbonate chemistry, inorganic nutrient concentrations, dissolved and particulate organic carbon concentrations etc.). Testing them in mesocosm enclosures has the additional benefit of minimizing public concern and regulatory requirements when compared to field trials.
Environmental impacts of OAE will be scale- and context-dependent in terms of the physical (e.g., timescales of mixing and CO2 equilibration, point source vs. diluted release), chemical (e.g., amount/type of alkaline substance, impurities), and biological characteristics (e.g., seasonal succession and related ecosystem vulnerability). Biological impacts are determined by exposure time and dose, ranging from acute shock responses on transient and local scales at point sources to chronic effects associated with possible transitions of ecosystem structure and performance at the regional and long-term scale. Key research questions which can be addressed adequately through mesocosm experiments are the following:
-
What is the safe operating space for OAE applications with respect to possible impacts on marine ecosystems functioning, biodiversity and ecosystem services?
-
How could OAE be implemented to reduce the risk of inadvertent negative environmental effects and maximize co-benefits?
-
Which biological indicators can serve as early warning signals or proxies for OAE environmental impacts?
-
How do different OAE approaches perform in terms of efficiency (e.g., mineral dissolution, CO2 uptake) and permanency (e.g., secondary precipitation)?
-
Which application sites are most appropriate for which OAE approach?
Mesocosm experiments offer a salient advantage over laboratory-based investigations, as they allow a realistic replication of natural communities. Multiple trophic levels can be confined under natural environmental conditions over a long period of time in a self-sustaining manner. Thereby, the same community can be sampled repeatedly over time. Furthermore, these experiments permit straightforward validation in the context of field research. Mesocosms, in essence, are closer to representing natural ecosystems characterized by carefully defined dimensions and monitored conditions and processes. To ensure realistic ecological boundary conditions, mesocosm experiments should be exposed to meteorological conditions resembling those of the target environment. Notably, the logistical flexibility of mesocosms affords researchers the opportunity to conduct investigations beyond the geographical confines of the environment under investigation. Consequently, mesocosms provide an invaluable avenue for the controlled study of specific environments and the impact of controlled manipulations therein. Given the diverse range of natural processes encountered in mesocosm experiments, external influences may be challenging to control, necessitating a robust monitoring strategy to achieve statistical power by either treatment replication or treatment gradients. Moreover, mesocosm experiments provide extensive multidisciplinary datasets that allow for a high degree of scientific integration and interdisciplinary collaboration. These datasets are valuable for parameterization and assessment of marine ecosystems and biogeochemical models.
While mesocosm experiments can be considered the preferred tool for the assessment of environmental impacts of OAE applications, they have several weaknesses that need to be considered when interpreting the data and extrapolating the results to the real world. These weaknesses include unnatural mixing and turbulence (in pelagic mesocosm), unnatural flow of bottom water across the sediment (in benthic mesocosms), wall effects and the growth of periphyton and other organisms on the mesocosm walls, spatial heterogeneity in the enclosed sediments, and the related difficulties in obtaining representative samples. The larger and more expensive the enclosures become, the more difficult it becomes to have a sufficient number of replicates in a replicated design or treatments in a gradient design. The fact that even the largest mesocosms enclose truncated communities (i.e., exclude higher trophic levels and highly migratory organisms) makes it difficult to adequately represent the responses of organisms with longer life cycles and the associated impacts on the food web. Another drawback of mesocosm experiments is their limited duration, due to the gradual diversion from their natural counterparts, e.g., due to community shifts, nutrient depletion and the consequent progressive loss of biological realism. The increasing variability between mesocosms in this process makes it increasingly difficult to identify treatment effects with statistical significance.
The primary purpose of a mesocosm experiment is to obtain “near-natural” conditions, that is to say, keeping the abiotic and biotic factors as close to the environment as possible in order to maximize the realism of the tested conditions. In general, timescale is related to mesocosm volume: the shorter the time needed for a controlled experiment, the smaller the enclosure size. Careful consideration should be given to the experimental design to adequately address the specific research questions and account for ecosystem- and site-specific characteristics as well as seasonal variability. The choice of the experimental configuration includes the three key dimensions of time, space and biological complexity, along with the required level of replication. Preference should be given to mimic the natural seasonal succession rather than provoking out-of-season events, e.g., triggering phytoplankton blooms through nutrient addition.
Considering the often limited number of experimental units, a critical consideration concerns the level of replication (Kreyling et al., 2018). The choice is between two basic approaches: (1) replicated (n ≥ 3) treatments, with limited treatment levels (e.g., Riebesell et al., 2007) and (2) a gradient approach with a larger number of non-replicated treatment levels (e.g., Taucher et al., 2017). The statistical power of the two options, using ANOVA statistics for the replicated design and regression statistics for the gradient design, is similar for the small number of experimental units typically available in mesocosm studies (Havenhand et al., 2010). If large within-treatment variation is expected, e.g., due to strong environmental variability or spatial heterogeneity, the replicated approach is recommended. In fact, strong within-treatment variability can easily mask subtle treatment effects. An important advantage of the gradient approach, on the other hand, is that it enables the identification of non-linearities, thresholds and tipping points in biological responses to OAE applications, relevant information for model parameterizations in terms of community functional responses. Knowledge about thresholds and possible tipping points is crucial also in the context of regulatory considerations for OAE implementation.
3.1 Pelagic mesocosms
When aiming to investigate OAE applications in the free water column, pelagic mesocosms are the research tool of choice. Among the various proposed strategies, ocean liming in the wake of ships would consist of sparging high-alkalinity fluids or mineral particles within the surface layer in offshore settings. In this scenario, any chemical perturbation is expected to affect in the first instance the pelagic domain and the planktic component of the marine ecosystem. Also OAE applications at fixed locations with a discharge of alkalinity-enriched water into coastal waters, e.g., desalination plants or sewage treatment plants, are best simulated in pelagic mesocosms. A suitable simulation of OAE approaches in which the alkalizing mineral is released in particulate form should ideally have the dissolution rate of the particles known in advance. If the rate is fast enough to ensure complete dissolution in the water column, pelagic mesocosms are well suited. In cases where the dissolution rate is slow compared to the particle sinking rate and particles sink to the seabed before dissolving, the experimental design may require a benthic component.
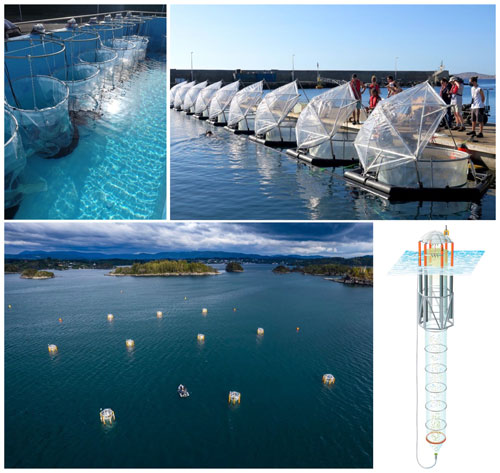
Figure 1Pelagic mesocosm facilities currently used in OAE research. Top left: land-based mesocosms (1 m3) at the University of Vigo, Spain. Top right: in situ on-shore mesocosms (10 m3) operated by GEOMAR, here employed on Gran Canaria, Spain. Bottom left: Kiel Off-Shore Mesocosms for Ocean Simulations (KOSMOS), here employed in the Raunefjord, Norway. Bottom right: sketch of a KOSMOS mesocosm unit (55 m3). Image sources: (top left) Daniela Basso, University of Milano-Bicocca; (top right) Ulf Riebesell, GEOMAR; (bottom left) Uli Kunz; (bottom right) Rita Erven, GEOMAR.
A missing component in all closed-system mesocosm experiments is the dilution through mixing with non-perturbed waters. Switching to an open system, where the enclosed water is partially replaced by non-alkalized water, places much greater demands on monitoring and complicates the interpretation of the observed responses, to the extent that it may be impossible to establish a reliable dose–response relationship. This experimental artifact is exacerbated when repeated additions of alkalinity are applied. Incorporating naturally occurring dilution in the experimental design can be done by applying the OAE treatment to only part of the enclosed water column and allowing for gradual mixing with the untreated water. The time until mixing can be controlled by stratifying the water column through a salinity gradient (adding fresh water into the upper layer or brine into the bottom layer, whereby the salinity change should be at a low enough level not to cause a biological response, e.g., a few tens of a salinity unit) or via a temperature stratification. Break-off of the stratification can be gradual or abrupt through active mixing. Parallel sampling of the OAE treated and untreated water bodies can provide insights about the compensating effect of dilution.
There is a wide range of enclosure volumes and structures used in pelagic mesocosm experimentation (Fig. 1). Among the various available solutions, the most obvious difference is the placement of the mesocosm: (1) stable, permanent structures on land or (2) floating bags in the water. All materials that come into contact with the enclosed water/sediment must be chemically inert; i.e., they must not leach or actively absorb any substances. Some technical details of the mesocosm design can markedly affect some abiotic factors, such as thermal characteristics, light conditions or mixing intensity of the enclosed water column. Most pelagic mesocosm enclosures are made of transparent material supported by a minimal rigid framework, with the intent to keep light conditions as in nature. Most materials, however, change the spectrum of the transmitted light; for example they are not transparent for UV light. As enclosure depth is often lower than the mixed-layer depth of the natural environment, natural light conditions are not well represented in mesocosms, with light intensities averaged over the mesocosm depth often higher than those averaged over the mixed-layer depth.
3.2 Benthic mesocosms
Benthic mesocosm experiments offer the unique chance to study OAE-mineral addition to the seafloor in a controlled setup. In comparison to experiments in laboratory settings, often small in scale with respect to mineral weathering, benthic mesocosms are more likely to mimic natural seafloor conditions and allow the coupling of biogeochemical processes at larger spatial and temporal scales. Key research questions on seabed alkalinization to be addressed in benthic mesocosm experiments include the following:
-
What are alkaline mineral dissolution rates under mesocosm conditions?
-
Do secondary minerals form that may compromise the net CO2 sequestration efficiency of this method?
-
How are microbial communities and macrofauna affected by mineral dissolution?
-
Is there a release and accumulation of heavy metals related to addition of silicate-based minerals and how does their toxicity affect the community/ecosystem?
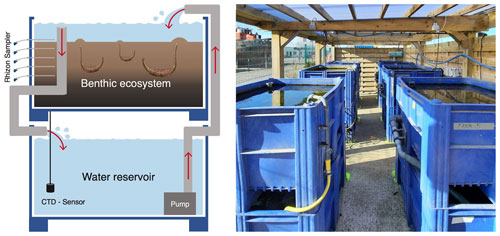
Figure 3In the benthic mesocosms at the University of Antwerp the dissolution kinetics of silicate minerals and the impacts on the benthic fauna in coastal environments have been monitored since 2019. The system comprises 20 units with two stacked tanks: the upper tank houses the benthic ecosystem, and the lower tank functions as a water reservoir. Natural sediment of 40 sediment heights with a mean grain size of 123 µm (3.0 ϕ) was collected from an intertidal sand flat in the Oosterschelde (the Netherlands) and mixed with olivine sand of similar grain size. Water from the Eastern Scheldt Estuary (salinity 32–35) is used to conduct flux sessions of 5 weeks (weekly sampling). At the end of each session, the total volume of water in each unit (∼ 500 L) is renewed (drawing: Astrid Hylén (UAnt); photo: Matthias Kreuzburg, https://www.coastal-carbon.eu/, last access: 7 November 2023, Geobiology, University of Antwerp).
Continuous water flow system. In this setup, a continuous flow of ambient seawater, preferably bottom water, over the sediment (Fig. 2), likely best resembles natural seafloor conditions. It is recommended to remove larger debris that could obstruct the water supply using a sediment trap (Fig. 2) whilst allowing small particulate matter to enter the mesocosms. The supply of particulate matter is essential to sustain natural microbial metabolism in the sediments and to provide food for filter-feeding macrofauna that colonize the sediment surface within a short period of weeks to months (Fig. 2). A relatively high flow rate is required (between 5000 to 10 000 L d−1) to keep the seawater well oxygenated and guarantee the survival of fauna and for maintaining the natural microbial communities as closely as possible to in situ conditions. With this setup, the bottom water should be monitored to trace seasonal changes in physical and chemical properties of the incoming seawater.
Water circulation approach. The benthic mesocosm setup with a seawater circulation approach consists of two tanks stacked on top of each other, with the upper tank housing the benthic ecosystem with sediments and organisms and the lower tank functioning as a seawater reservoir from which water is pumped into the upper tank (Fig. 3). Thus, a constant flow of water is generated through the water in- and outflow, and the height of the water column in the upper tank can be controlled by the vertical positioning of the outflow. The tanks for the benthic mesocosms have a volume of approximately 1 m2 and are situated outdoors and exposed to natural temperature fluctuations.
Based on the water circulation approach, the closed system allows for the detection and accumulation of weathering products and to focus on a specific process or reaction, such as the dissolution kinetics of silicate minerals in the case of the University of Antwerp study (Fig. 3). After a defined time span (flux session) the total amount of water is replaced and accumulation of weathering products starts again from initial values. In terms of this experiment design, ≥ 3 replicates of benthic mesocosms are crucial to ensure that results are statistically significant and can be generalized to the broader ecosystem being studied (e.g., Wadden Sea).
The total experiment duration as well as the sampling strategy is defined by the research questions, and longer experiments may be necessary to capture seasonal or long-term trends in the system. The use of natural sediment and the inclusion of a dominant bioturbating organism (e.g., Arenicola marina) in benthic mesocosm experiments is a crucial step toward making the experimental setup more representative of real-world conditions. However, it is important to emphasize that the choice of sediment type and benthic organisms should be aligned with the specific research objectives and questions being addressed.
In OAE studies involving benthic mesocosms, various types of sediments can be considered, ranging from fine-grained sediments to rocky substrates. The selection of sediment type should be guided by factors such as the local environmental conditions, the availability of sediment types that reflect the targeted ecosystem and the specific geochemical interactions being investigated. For studies related to carbonate dissolution and alkalinity enhancement as given above, fine-grained or sandy sediments are most suitable, given their potential to facilitate mineral dissolution and subsequent alkalinity release.
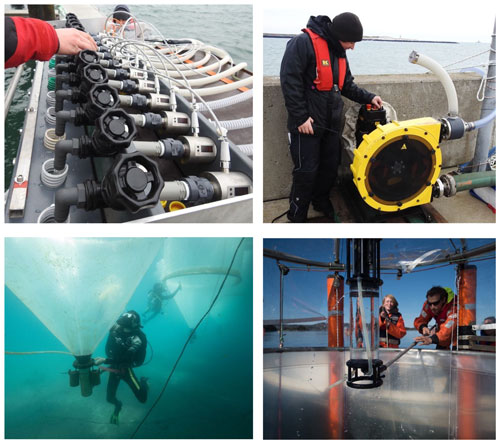
Figure 4Upper left: distributor control system enabling parallel filling of all mesocosms. Upper right: peristaltic pump ensuring smooth flow of source water during filling of the mesocosms, keeping damage to fragile organisms at a minimum. Lower left: sediment traps forming the bottom of in situ mesocosm enclosures. Lower right: programmable water sampler, enabling depth-integrated water samples over the entire mesocosm depth (or parts thereof) (photo sources: (upper left and upper right) Ulf Riebesell; (lower left) Michael Sswat; (lower right) Solvin Zankl).
Similarly, the choice of benthic organisms should be tailored to the research objectives. While many benthic organisms can be tested in mesocosms, it is important to consider the life history, behavior and ecological role of the selected species (Bach et al., 2019; Flipkens et al., 2021). For instance, if the experiment spans a year and aims to study the recruitment and life cycle of benthic organisms that have a pelagic phase, careful planning is required. Monitoring larval settlement, growth and interactions with the sediment during their benthic phase becomes integral to such investigations.
As an illustrative example, consider an OAE study targeting the enhancement of carbonate precipitation through the addition of alkalinity. In a coastal setting, sandy sediments rich in carbonate minerals might be chosen, given their potential for mineral dissolution and subsequent bicarbonate formation. Benthic organisms like filter-feeding mollusks and burrowing polychaetes could be tested to assess their responses to altered alkalinity levels.
Finally, the water circulation approach should be carefully designed to ensure consistency in water flow rates and initial seawater chemistry. Sedimentation in the water reservoir tank has to be prevented to avoid secondary sediment surfaces, and a continuous monitoring system (salinity, temperature) is recommended to estimate evaporation rates. In addition, regular sampling of environmental conditions (humidity, pCO2) as well as carbonate system parameters and nutrients can ensure that the experiment proceeds as planned and that the results are reliable.
Filling of the mesocosms is a delicate process that, if not done with care, can jeopardize the entire experiment. A key aspect is to ensure identical starting conditions, both for the abiotic and biotic conditions in all mesocosms. Between-mesocosm differences in baseline conditions can cause divergence of the enclosed communities and severely hamper the detection of treatment effects. As the filling often represents a major perturbation itself, some time of equilibration may be needed before applying the treatment manipulation and starting the actual experiment. The time for equilibration may differ for pelagic and benthic habitats as well between different ecosystems and seasons. Adequate monitoring during this pre-manipulation phase can determine when a new steady state is reached and confirm whether all mesocosms have similar starting conditions. Key parameters for which equal starting conditions among mesocosms need to be ensured include temperature, salinity, inorganic nutrient concentrations, the carbonate chemistry (pH, pCO2, dissolved inorganic carbon, DIC, and total alkalinity, TA) dissolved and particulate organic matter concentrations, community composition and diversity, and standing stocks of the dominant taxonomic groups across trophic levels.
Another critical aspect of mesocosm operation is taking representative samples. The enclosed water bodies and sediments typically show spatial heterogeneity (vertical gradients in the water column and sediments, patchiness in the distribution of larger organisms). The spatial variability of the target variables of the enclosed system should be determined prior to deciding on the best sampling strategy. Sampling bias related to vertical gradients, e.g., water column nutrient concentration and phytoplankton biomass, can be overcome by taking depth-integrated water samples (Fig. 4). Some species may even perform diurnal vertical migration, which also should be accounted for in the sampling strategy.
Mesocosm enclosures are always associated with additional surfaces, the mesocosm walls, that are not present in the natural environment. The smaller the mesocosms, the larger the additional surface area relative to the enclosed volume. Free surfaces are generally subject to rapid biofilm formation, followed by colonization of larger organisms. The associated microbial community can significantly influence water column processes, which is of particular concern in pelagic mesocosms. To minimize such wall effects, cleaning of the mesocosm walls can be useful. Specific to OAE mesocosm experimentation is that under conditions where the water column is highly oversaturated with respect to calcium carbonate, mesocosm walls can provide free surfaces for secondary precipitation of carbonates. Under these circumstances, wall cleaning can scrape off these carbonates, creating additional precipitation nuclei in the water column. If wall cleaning is continued under these circumstances, possible effects caused by this, e.g., enhancement of secondary precipitation in the water column and increased ballasting of particulate matter, should be seen as artifacts and interpreted as such. If wall cleaning is discontinued and the biofilm on the walls grows to a significant biomass compared to the suspended biomass, this may limit the duration of the experiment. The decision for or against wall cleaning must be made on a case-by-case basis and depends, among other things, on the severity of wall growth, the duration of the experiment and the specific research questions to be investigated.
4.1 Pelagic mesocosms
Different techniques have been employed for filling pelagic mesocosms, including (1) direct pumping from the sea in cases where mesocosms are placed in situ or close to natural waters, (2) collection in tanks when source waters need to be transported over some distance and subsequent pumping from the tanks into the mesocosm, and (3) lowering a flexible bag like a curtain over an undisturbed water column. In all cases care should be taken to fill the mesocosms with identical source waters. Considering that water masses may change over the filling procedure, this can best be achieved by filling the mesocosms in parallel through a distributor system (Fig. 4). Likewise, if several tanks are needed to obtain the required source water volume, the water of each tank should be distributed evenly into all mesocosm units. The source water should be representative of the targeted ecosystem. This concerns the depth at which the source water is collected and, when diurnally vertically migrating organisms are present, the time of day. When pumping is applied some damage to fragile organisms, e.g., gelatinous zooplankton, is unavoidable. It is therefore recommended to use pumps that ensure a smooth flow of pumped water, e.g., peristaltic pumps (Fig. 4). To prevent large and rare organisms from entering and being unevenly distributed in the mesocosms, some screening can be applied at the intake of the pumping hose.
As mentioned above a typical artifact of mesocosm enclosures is the reduced level or absence of turbulence. In mesocosms with solid wall structures it may be useful to apply some form of mixing of the water column, considering that turbulence (including its absence) is known to strongly affect the plankton community composition and succession. In floating enclosures with flexible walls some turbulence is induced by surface wave action, below surface water movement and variability in water currents, but the vorticity of the enclosed water is still always much reduced compared to that of the natural environment. Somewhat related to the mixing regime is another potential artifact in mesocosms where settling particulate matter is continuously resuspended from the bottom. Resuspension of degrading organic matter, which under natural conditions would sink out of the upper mixed layer, exaggerates the heterotrophic processes in the system. Collecting and removing the sedimented matter in cone-shaped sediment traps which form the bottom of the mesocosms can avoid this problem (Fig. 4).
4.2 Benthic mesocosms
A particular challenge in benthic mesocosm experiments concerns the filling with sediment from the seafloor. Depending on the size of the tanks and the sediment height, it may be necessary to transfer several hundreds of kilograms of sediment from the seafloor to the tanks. Near-intact sediments (undisturbed vertical stratification) may be collected relatively easily in sub-tidal areas. At sea, undisturbed sediments may be retrieved using a box corer or similar device, although this may be a tedious exercise involving multiple deployments of the coring equipment. Large amounts of sediment can be gathered relatively easily and quickly using a sediment grab, but disturbance of the sediment matrix is inevitable, and longer equilibration times for the sediment geochemistry to stabilize will be required before experiments can be started. In any case, benthic communities within mesocosms may be altered from those in natural ecosystems, and a sound understanding of the equilibration period is crucial to allow for changes in benthic communities and the establishment of a new steady state within the benthic mesocosm. This equilibration period should be determined based on the specific conditions of the mesocosm experiment, including the number of replicates, environmental parameters and the selected organisms. Adequate monitoring and sampling during the equilibration period are essential to ensure that the experimental conditions have stabilized and the ecosystem has reached a new steady state, which in turn increases material and labor requirements. Robust control units are crucial in benthic mesocosm experiments and should ideally consist of the same number of replicates as the treatment group to ensure that any observed changes are due to the experimental treatments rather than natural variability. Sampling and monitoring should be in the same manner as the treatment group.
Different minerals, waste materials and electrochemical products have been suggested as feedstock for ocean alkalinity enhancement (for a comprehensive introduction to potential source materials, see Eisaman et al., 2023, this Guide). Most source materials do not come as pure alkalinity but rather contain other substances, such as silicate, calcium, magnesium and various trace metals (e.g., iron, nickel, cobalt, chromium). OAE can be achieved by addition in dissolved form, which requires dissolution of the feedstock before its release into the sea, or in particulate form, after grinding of the feedstock, with the grain size being one important factor determining the dissolution rate. OAE can further be conducted in a CO2-equilibrated mode, which involves some form of active injection of CO2 into the alkalinity-enriched source water prior to its release, or in a non-equilibrated mode, which relies on air–sea gas exchange to provide the additional CO2 that the alkalinized seawater can absorb. In the case of the latter it is important to keep in mind that the timescales for CO2 equilibration are on the order of months and can only occur as long as the alkalinized seawater is in contact with the atmosphere (see Schulz et al., 2023, this Guide, for further details).
Taken together, this results in a wide range of possible application scenarios, not all of which can be tested with the same scrutiny in mesocosm experiments due to the high financial and personnel costs involved. Hence, it is important to focus on those OAE application scenarios which are most likely to be implemented. As the field of OAE R&D is developing rapidly and dynamically, there will likely be changes in what is considered the most suitable OAE application approaches, in terms of cost, efficiency, environmental safety, friendliness in terms of monitoring, verification and reporting (MRV), technological readiness, and the regulatory requirements for their implementation. Mesocosm research in this field should maintain sufficient flexibility to respond to those changes and aim for testing “real-world” scenarios of OAE applications. On the other hand, because the results obtained from mesocosm studies will likely be context-specific (depending on, e.g., ecosystem type, time of year, latitudinal location, hydrographic setting) and depend on the mesocosm setup and operation itself, it takes multiple such studies for a given OAE approach to reach robust conclusions about its environmental safety. To facilitate inter-comparison between results, it would be favorable to use standardized mesocosms and follow common protocols for mesocosm experimentation.
From an experimental perspective, there is a trade-off between testing pure alkalinity enhancement and feedstocks which involve the release of other biologically active components. While the latter is more in line with real-world applications, it complicates the interpretation of the observed responses due to confounding factors and limits the extrapolation of the findings, considering that the stoichiometric composition differs between feedstocks. As the field is currently still at an early stage and considering that the number of mesocosm studies will likely be small due to their high costs, it seems beneficial to first establish a basic understanding of alkalinity effects in isolation, before turning to more feedstock-specific testing. This being said, we note that the above-mentioned confounding effects may actually be the intended research question or that the focus may be on a specific feedstock likely to be utilized widely. In general, we recommend designing mesocosm experiments with a more generic approach first and addressing feedstock-specific in smaller-scale laboratory-based experiments.
5.1 Pelagic mesocosms
Alkalinity manipulations in pelagic mesocosms are fairly straightforward when done in dissolved form. Dissolving the alkaline feedstock in freshwater or deionized water prevents secondary carbonate precipitation during preparation of the concentrated solution (we note that the use of freshwater for feedstock dissolution may not be practical for large-scale implementation of OAE). To avoid confounding effects of the freshwater addition on the mesocosm community, the volume should be kept to a minimum. Using source materials with a high solubility in water, such as NaHCO3, Na2CO3, Ca(OH)2 or NaOH, enables highly concentrated alkaline source water (Hartmann et al., 2023). To simulate CO2-equilibrated alkalinization, NaHCO3 and Na2CO3 can be combined in appropriate proportions (Subhas et al., 2022); for non-equilibrated alkalinization, carbonate-free source materials such as NaOH and Ca(OH)2 can be used (Moras et al., 2022). To avoid prolonged pH peaks and secondary precipitation during the injection procedure, it needs to be assured that the concentrated solution is mixed in rapidly. One way to achieve a uniform alkalinity enhancement across the water column is to move a distribution device with multiple outlets up and down the mesocosms at a constant speed (Fig. 5). Flocculent precipitates that form directly at the injection site are usually not stable and disappear quickly when further diluted through mixing. Care should be taken to ensure that the added alkalinity is evenly distributed throughout the enclosed water column.
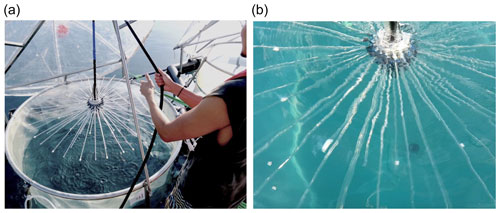
Figure 5(a) Distribution device used for alkalinity addition; by moving it up and down in the water column during alkalinity injection at constant speed, a uniform alkalinity enhancement can be achieved. (b) Milky water at the outlet of the injection tubes indicates temporary precipitation, which, however, quickly disappears as the highly concentrated alkalinity solution dilutes. Photo sources: Ulf Riebesell.
Alkalinity enhancement in particulate form is far less practical. If the particles sink faster than they dissolve, they accumulate on the mesocosm floor or sink directly into the trap in mesocosms with a sediment trap at the bottom. Accumulation and subsequent dissolution at the bottom might lead to highly concentrated alkalinity enrichment, enhancing the risk of secondary precipitation and of strong negative impacts in bottom waters. Alkaline particles sinking into the sediment trap would be lost from the mesocosm enclosure during the next trap sampling. In both cases it would be considered an experimental artifact. It is therefore recommended to use minerals with high dissolution rates (e.g., NaOH, CaO, Ca(OH)2,Mg(OH)2) and small grain sizes to ensure dissolution before the mineral particles reach the bottom of the mesocosms (see Eisaman et al., 2023, this Guide, for a detailed description of technical aspects of OAE).
Monitoring of seawater carbonate chemistry in the water column should adhere to the guidelines provided in Schulz et al. (2023, this Guide). High levels of non-equilibrated alkalinization can lead to secondary precipitation, triggering a process termed “runaway precipitation” (Moras et al., 2022; Hartmann et al., 2023), whereby carbonate formation can consume more alkalinity than initially added. It seems that the initiation of this process can occur both in the water column and on the mesocosm walls. As the carbonate crystals grow in size, their sinking velocity increases. When incorporated in organic matter aggregates they serve as ballast, thereby increasing the vertical flux of organic matter. In addition, carbonate crystals could affect mobility and feeding of plankton organisms, with possible adverse effects on food web interactions and trophic transfer. Secondary precipitation also increases seawater turbidity, affecting light attenuation and possibly primary production. Collecting this sinking particulate matter in sediment traps at the bottom of the mesocosms enables the quantification and identification of the precipitates and provides information about the chemical reactions leading to their formation. In mesocosms without integrated sediment traps, simple traps can easily be set up on the bottom and sampled through a tube that reaches the surface.
5.2 Benthic mesocosms
Alkalinity enhancement in the benthic mesocosm approach is achieved by mineral addition, which dissolves in the surface sediment over time. In general, the addition of sedimentary OAE source materials (e.g., siliciclastic minerals, carbonates; Eisaman et al., 2023, this Guide) modifies the grain size distribution of the sediment and thus affects the porosity, permeability and water flow through the sediment. The changing sediment structure can impact living conditions for organisms, as well as the distribution and abundance of organisms living in the sediment and their behavior and ecology. With respect to mineral addition, the grain size selection is important, as a trade-off between grain size and production costs is required (e.g., Hartmann et al., 2013). Previous studies have investigated the relationship between CO2 sequestration efficiency and grain sizes, and there is a general assumption that small grain sizes reveal higher dissolution rates and CO2 sequestration rates due to larger reactive surface areas, whereas more grinding energy is required generating a higher CO2 footprint and lower CO2 sequestration efficiencies (Köhler et al., 2010; Renforth and Henderson, 2017; Foteinis et al., 2023). Clearly, the CO2 emissions during production and transport must be significantly lower than the potential CO2 sequestration of benthic mineral dissolution (see Eisaman et al., 2023, this Guide). The selection of appropriate grain sizes for the addition of alkaline minerals is a critical consideration for experimental studies, particularly in the context of the target environment's geological setting. From an environmental perspective, it is recommended to choose comparable grain sizes that are stable under in situ hydrodynamic conditions. For highly dynamic ecosystems such as the Wadden Sea, estuaries and wave-dominated coastal areas, a range of grain sizes from fine to coarse sand (0.075 to 2 mm) may be appropriate for experimental approaches. However, in low-dynamic systems such as lagoons, enclosed bays or shelf regions, grain sizes from silt to very fine sand (< 0.075 mm) can be considered for investigation. This approach would also help to ensure that the sedimentary structure and settings for organisms in the mesocosms are representative of the natural conditions of the target environment.
It may be practical to interrupt the water circulation system during mineral deployment in order to allow sedimentation of the suspended matter. To achieve a uniform alkalinity enhancement in the benthic mesocosms, minerals should be evenly distributed. To induce a measurable effect on alkalinity changes in the envisioned experimental time, grain sizes smaller than 1 mm are desirable (Strefler et al., 2018). The addition to the marine environment could best be achieved through a mixture of natural seawater, marine sediments and OAE source materials. This may ensure a more uniform distribution and reduce the purity of industrially produced OAE source materials, which are poor in nutrients and microbial organisms. Thus, this approach is also recommended for the addition of silicates to benthic mesocosms. By using a mixture, the potential effects of silicate addition can be more accurately evaluated because the experimental conditions are more similar to those in the natural environment.
For calcium carbonate, it may be reasonable to use the annual flux of particulate organic matter to the seafloor as an upper estimate of the required mineral to be added. The underlying assumption here is that the added mineral can completely neutralize the natural CO2 produced from organic matter degradation. However, this assumes that mineral dissolution efficiency is close to 100 %, which may not be the case if it is mixed below the undersaturated layers. Adding minerals in large excess risks clogging the surface layer and creating a physical barrier against effective benthic–pelagic coupling of solute fluxes. Finding the optimal mineral dosage to achieve a balance between dissolution efficiency and dissolution rate would likely be specific to the local environmental characteristics and require testing at each potential mineral addition site. For silicate minerals (e.g., olivine), the upper limit of mineral addition per square meter will also depend on the trace metal concentrations (Flipkens et al., 2021). Based on the variation in Ni content of marine sediments (prior to the addition of olivine), this implies that the allowable range for the addition of olivine is between 0.059 and 1.4 kg m2 of seafloor without posing a risk to benthic biota. This threshold is based on Environmental Quality Standards (EQS), which are derived from metal toxicity data using methods such as species sensitivity distributions (SSDs). They provide threshold metal concentrations in seawater or sediment that are considered protective for the aquatic environment and are used by industries, governments and environmental agencies to guide regulations. So far, these guidelines have been only appropriate to specific regions and environments and may need to be re-evaluated for broader use in OAE applications.
Monitoring of mineral dissolution will be determined by the experimental design. A major drawback of a high throughflow is that rapid dilution and flushing of geochemical tracers emitted from the sediment compromises the analytical detection of dissolving alkaline minerals in the overlying water and the reliable assessment of the effectiveness of the method. In this case, alternative ways of mineral dissolution detection may be required. For instance, alkalinity enhancement may be detectable in pore fluids, which can be extracted using filters (e.g., rhizons) inserted horizontally through holes pre-drilled vertically in the tank (Fig. 6). However, the vertical sampling resolution may be too coarse to detect mineral dissolution close to the sediment surface. Microelectrodes for O2, pH and H2S are arguably a better alternative to detect changes in surface geochemistry in the uppermost centimeters after mineral addition. An advantage of the high dilution factors is the potential suppression of secondary mineral formation such as phyllosilicates and/or carbonates, which could reduce the net CO2 sequestration efficiency of OAE (Fuhr et al., 2022; Moras et al., 2022; Hartmann et al., 2023). Secondary mineral formation is a common process in marine seafloor sediments, potentially impacting global carbon and element cycles on a global scale, and the controlling factors have not been unambiguously identified to date (e.g., Rahman et al., 2017; Torres et al., 2020; Geilert et al., 2023).
The deployment of benthic incubation chambers within the mesocosms themselves is a non-invasive method for detecting alkalinity release following mineral addition (Fig. 6). These benthic chambers enclose a certain area of the surface sediment and allow the accumulation of alkalinity and other components of interest over time, from which benthic fluxes can be determined. Mineral dissolution rates can be estimated by comparison with control mesocosms where no minerals were artificially added. Fluid sampling can be achieved by hand via suction using connected tubing and syringes. Care is needed to prevent hypoxia or anoxia inside the chambers due to respiration by benthic biota, which may be observable by a blackening of the sediment surface due to precipitation of iron sulfide minerals. Low oxygen levels will result in an interruption to the normal respiration rates of animals causing them to resurface. This may alter natural sediment mixing rates as well as mineral saturation states via changes in biogeochemical turnover rates and pathways in the sediment. Together, these undesired artifacts may be reflected in unrealistic fluxes of alkalinity and other solutes from the sediment. Completely interrupting the water flow to the whole benthic mesocosm in order to detect changes in bottom water alkalinity will only serve to magnify these side effects.
Recommendations
General recommendations include the following:
-
Use inert materials for mesocosm hardware (e.g., plastics, stainless steel).
-
Select the mesocosm size and experimental duration according to the enclosed community and processes studied.
-
Choose the experimental design to maximize the statistical power and report it.
-
Maximize similarity in starting conditions between mesocosms during enclosure filling.
-
Monitor starting conditions before applying experimental treatment.
-
Allow for the natural (e.g., seasonal) succession and avoid out-of-season events.
-
Avoid confounding factors and perturbations other than the intended treatments.
-
Adapt the sampling frequency to the dynamics of the processes studied.
-
Determine spatial heterogeneity and take account of it in the sampling strategy.
-
Apply depth-integrated sampling in case of vertical gradients (pelagic mesocosms).
-
Minimize wall growth, e.g., by regularly cleaning the walls.
OAE-specific recommendations include the following:
-
Test real-world OAE scenarios, focusing on those most likely to be implemented.
-
Keep some flexibility to respond to changes in the OAE R&D field.
-
Monitor carbonate chemistry with at least two carbonate system parameters and watch out for secondary precipitation.
-
Maximize transferability of results by testing generic OAE approaches.
-
Take note of the context specificity of the observed ecosystem responses.
-
Provide detailed information of the feedstock composition utilized for experimental manipulations.
-
Closely monitor signs of potential barriers to OAE implementation (e.g., long-term restructuring of community composition and functioning, decline in ecosystem productivity, proliferation of harmful species, disruption of trophic transfer, changes in elemental cycling).
No data sets were used in this article.
UR scoped and edited the contents of the manuscript. UR drafted the general text, with contributions from all co-authors. UR drafted the sections specific to pelagic mesocosms, with contributions from DB. SG, AWD and MK drafted the sections specific to benthic mesocosms. All authors contributed to revising the manuscript.
Competing interests are declared in a summary for the entire volume at: https://sp.copernicus.org/articles/sp-oae2023-ci-summary.zip.
Publisher's note: Copernicus Publications remains neutral with regard to jurisdictional claims made in the text, published maps, institutional affiliations, or any other geographical representation in this paper. While Copernicus Publications makes every effort to include appropriate place names, the final responsibility lies with the authors.
We thank the Ocean Acidification and other ocean Changes – Impacts and Solutions (OACIS), an initiative of the Prince Albert II of Monaco Foundation, for its support throughout the project. We extend our gratitude to the Villefranche Oceanographic Laboratory for supporting the meeting of the lead authors in January 2023. Ulf Riebesell acknowledges funding from the European Union's Horizon 2020 Research and Innovation Program under grant 869357 (project OceanNETs: Ocean-based Negative Emission Technologies analyzing the feasibility, risks, and co-benefits of ocean-based negative emission technologies for stabilizing the climate). Daniela Basso acknowledges funding from the Prince Albert II of Monaco Foundation for the OACIS project “Ocean alkalinity enhancement: a mesocosm-scale approach”. Sonja Geilert and Ulf Riebesell acknowledge funding from the German Federal Ministry of Education and Research (Grant No. 03F0895) Project RETAKE, DAM Mission “Marine carbon sinks in decarbonization pathways” (CDRmare).
This research has been supported by the ClimateWorks Foundation (grant no. 22-0296) and the Prince Albert II of Monaco Foundation. It has also been supported by the European Union's Horizon 2020 Research and Innovation Program (project OceanNETs: Ocean-based Negative Emission Technologies analyzing the feasibility, risks, and co-benefits of ocean-based negative emission technologies for stabilizing the climate; grant no. 869357), the Prince Albert II of Monaco Foundation (OACIS project “Ocean alkalinity enhancement: a mesocosm-scale approach”), and the German Federal Ministry of Education and Research (Project RETAKE, DAM Mission “Marine carbon sinks in decarbonization pathways” (CDRmare), grant no. 03F0895).
The article processing charges for this open-access publication were covered by the GEOMAR Helmholtz Centre for Ocean Research Kiel.
This paper was edited by Rosalind Rickaby and reviewed by Behzad Mostajir, Alex Poulton, and one anonymous referee.
Bach, L. T., Gill, S. J., Rickaby, R. E. M., Gore, S., and Renforth, P.: CO2 Removal With Enhanced Weathering and Ocean Alkalinity Enhancement: Potential Risks and Co-benefits for Marine Pelagic Ecosystems, Front. Clim., 1, 7, https://doi.org/10.3389/fclim.2019.00007, 2019.
Eisaman, M. D., Geilert, S., Renforth, P., Bastianini, L., Campbell, J., Dale, A. W., Foteinis, S., Grasse, P., Hawrot, O., Löscher, C. R., Rau, G. H., and Rønning, J.: Assessing the technical aspects of ocean-alkalinity-enhancement approaches, in: Guide to Best Practices in Ocean Alkalinity Enhancement Research, edited by: Oschlies, A., Stevenson, A., Bach, L. T., Fennel, K., Rickaby, R. E. M., Satterfield, T., Webb, R., and Gattuso, J.-P., Copernicus Publications, State Planet, 2-oae2023, 3, https://doi.org/10.5194/sp-2-oae2023-3-2023, 2023.
Flipkens, G., Blust, R., and Town, R. M.: Deriving Nickel (Ni(II)) and Chromium (Cr(III)) based environmentally safe olivine guidelines for coastal enhanced silicate weathering, Environ. Sci. Technol., 55, 12362–12371, https://doi.org/10.1021/acs.est.1c02974, 2021.
Foteinis, S., Campbell, J. S., Renforth, P.: Life cycle assessment of coastal enhanced weathering for carbon dioxide removal from air, Environ. Sci. Technol., 57, 6169-6178, https://doi.org/10.1021/acs.est.2c08633, 2023.
Fuhr, M., Geilert, S., Schmidt, M., Liebetrau, V., Vogt, C., Ledwig, B., and Wallmann, K.: Kinetics of olivine weathering in seawater: An experimental study, Front. Clim., 4, 1–20, https://doi.org/10.3389/fclim.2022.831587, 2022.
Geilert, S., Frick, D. A., Garbe-Schönberg, D., Scholz, F., Sommer, S., Grasse, P., Vogt, C., and Dale, A. W.: Coastal El Niño triggers rapid marine silicate alteration on the seafloor, Nat. Commun., 14, 1676, https://doi.org/10.1038/s41467-023-37186-5, 2023.
Hartmann, J., West, A. J., Renforth, P., Köhler, P., De La Rocha, C. L., Wolf-Gladrow, D. A., Dürr, H. H., and Scheffran, J.: Enhanced chemical weathering as a geoengineering strategy to reduce atmospheric carbon dioxide, supply nutrients, and mitigate ocean acidification, Rev. Geophys., 51, 113–149, https://doi.org/10.1002/rog.20004, 2013.
Hartmann, J., Suitner, N., Lim, C., Schneider, J., Marín-Samper, L., Arístegui, J., Renforth, P., Taucher, J., and Riebesell, U.: Stability of alkalinity in ocean alkalinity enhancement (OAE) approaches – consequences for durability of CO2 storage, Biogeosciences, 20, 781–802, https://doi.org/10.5194/bg-20-781-2023, 2023.
Havenhand, J., Dupont, S., and Quinn, G.: Designing ocean acidification experiments to maximise inference, in: Guide for best practices for ocean acidification research and data processing, edited by: Riebesell, U., Fabry, V. J., Hansson, L., and Gattuso, J.-P., Publications Office of the European Union, Luxembourg, 258 pp., https://doi.org/10.2777/66906, 2010.
Köhler, P., Hartmann, J., and Wolf-Gladrow, D. A.: Geoengineering potential of artificially enhanced silicate weathering of olivine, P. Natl. Acad. Sci. USA, 107, 20228–20233, https://doi.org/10.1073/pnas.1000545107, 2010.
Kreyling, J., Schweiger, A. H., Bahn, M., Ineson, P., Migliavacca, M., Morel-Journel, T., Christiansen, J. R., Schtickzelle, N., and Larsen, K. S.: To replicate, or not to replicate – that is the question: How to tackle nonlinear responses in ecological experiments, Ecol. Lett., 21, 1629–1638, https://doi.org/10.1111/ele.13134, 2018.
Moras, C. A., Bach, L. T., Cyronak, T., Joannes-Boyau, R., and Schulz, K. G.: Ocean alkalinity enhancement – avoiding runaway CaCO3 precipitation during quick and hydrated lime dissolution, Biogeosciences, 19, 3537–3557, https://doi.org/10.5194/bg-19-3537-2022, 2022.
Rahman, S., Aller, R. C., and Cochran, J. K.: The missing silica sink: Revisiting the marine sedimentary Si cycle using cosmogenic 32Si, Global Biogeochem. Cy., 31, 1559–1578, https://doi.org/10.1002/2017GB005746, 2017.
Renforth, P. and Henderson, G.: Assessing ocean alkalinity for carbon sequestration, Rev. Geophys., 55, 636–674, https://doi.org/10.1002/2016RG000533, 2017.
Riebesell, U., Schulz, K. G., Bellerby, R. G. J., Botros, M., Fritsche, P., Meyerhöfer, M., Neill, C., Nondal, G., Oschlies, A., Wohlers, J., and Zöllner, E.: Enhanced biological carbon consumption in a high CO2 ocean, Nature, 450, 545–549, https://doi.org/10.1038/nature06267, 2007.
Riebesell, U., Fabry, V. J., Hansson, L., and Gattuso, J.-P.: Guide for best practices for ocean acidification research and data processing, Publications Office of the European Union, Luxembourg, 258 pp., https://doi.org/10.2777/66906, 2010.
Schulz, K. G., Bach, L. T., and Dickson, A. G.: Seawater carbonate chemistry considerations for ocean alkalinity enhancement research: theory, measurements, and calculations, in: Guide to Best Practices in Ocean Alkalinity Enhancement Research, edited by: Oschlies, A., Stevenson, A., Bach, L. T., Fennel, K., Rickaby, R. E. M., Satterfield, T., Webb, R., and Gattuso, J.-P., Copernicus Publications, State Planet, 2-oae2023, 2, https://doi.org/10.5194/sp-2-oae2023-2-2023, 2023.
SCOR Working Group 85: Manual on marine experimental ecosystems, 2nd report, UNESCO technical papers in marine science, Paris, 178 pp., ISSN 0503-4299, https://www.jodc.go.jp/jodcweb/info/ioc_doc/UNESCO_tech/090201eb.pdf (last access: 8 November 2023), 1991.
Stewart, R. I. A., Dossena, M., Bohan, D. A., Jeppesen, E., Kordas, R. L., Ledger, M. E., Meerhoff, M., Moss, B., Mulder, C., Shurin, J. B., Suttle, B., Thompson, R., Trimmer, M., and Woodward G.: Mesocosm experiments as a tool for ecological climate-change research, in: Adv. Ecolog. Res., edited by: Woodward, G. and O'Gorman, E. J., Academic Press, Amsterdam, the Netherlands, 48, 71–181, https://doi.org/10.1016/B978-0-12-417199-2.00002-1, 2013.
Strefler, J., Amann, T., Bauer, N., Kriegler, E., and Hartmann, J.: Potential and costs of carbon dioxide removal by enhanced weathering of rocks, Environ. Res. Lett. 13, 034010, https://doi.org/10.1088/1748-9326/aaa9c4, 2018.
Subhas, A. V., Marx, L., Reynolds, S., Flohr, A., Mawji, E. W., Brown, P. J., and Cael, B. B.: Microbial ecosystem responses to alkalinity enhancement in the North Atlantic Subtropical Gyre, Front. Clim., 4, 784997, https://doi.org/10.3389/fclim.2022.784997, 2022.
Taucher, J., Bach, L. T., Boxhammer, T., Nauendorf, A., The Gran Canaria KOSMOS Consortium, Achterberg, E. P., Algueró-Muñiz, M., Arístegui, J., Czerny, J., Esposito, M., Guan, W., Haunost, M., Horn, H. G., Ludwig, A., Meyer, J., Spisla, C., Sswat, M., Stange, P., and Riebesell, U.: Influence of ocean acidification and deep water upwelling on oligotrophic plankton communities in the Subtropical North Atlantic: Insights from an in situ mesocosm study, Front. Mar. Sci., 4, 85, https://doi.org/10.3389/fmars.2017.00085, 2017.
Torres, M. E., Hong, W.-L., Solomon, E. A., Milliken, K., Kim, J.-H., Sample, J. C., Teichert, B. M. A., and Wallmann, K.: Silicate weathering in anoxic marine sediment as a requirement for authigenic carbonate burial, Earth-Sci. Rev., 200, 102960, https://doi.org/10.1016/j.earscirev.2019.102960, 2020.
- Abstract
- Preface
- Placing mesocosms in the context of OAE research
- Strengths and weaknesses of mesocosm experimentation
- Experimental design
- Mesocosm operation: filling, sampling, wall cleaning
- Alkalinity manipulation and monitoring
- Data availability
- Author contributions
- Competing interests
- Disclaimer
- Acknowledgements
- Financial support
- Review statement
- References
- Abstract
- Preface
- Placing mesocosms in the context of OAE research
- Strengths and weaknesses of mesocosm experimentation
- Experimental design
- Mesocosm operation: filling, sampling, wall cleaning
- Alkalinity manipulation and monitoring
- Data availability
- Author contributions
- Competing interests
- Disclaimer
- Acknowledgements
- Financial support
- Review statement
- References